Chapter 20: Advancement and Utilization of Biosensors for Protein Interaction Analysis and Proteomics
Simon Cocklin,1 Tetsuya Ishino,1 Mauro Sergi,1 Dobrin Nedelkov,2 Randall W. Nelson,2 and Irwin Chaiken1
1Biochemistry Department and the A.J. Drexel Institute of Basic and Applied Protein Science, Drexel University College of Medicine, Philadelphia, Pennsylvania 19102; 2Intrinsic Bioprobes Inc., Tempe, Arizona 85281
INTRODUCTION: IN THE PURSUIT OF PROTEIN–PROTEIN INTERACTIONS AND INTERACTION NETWORKS
Progress in genomics and proteomics is leading to the complete identification of the proteins that drive virtually all biological processes (Anderson et al. 2000; Howard 2000; Dongre et al. 2001). The networks of interactions between proteins that control cell function in living organisms are beginning to be revealed (Giot et al. 2003). Along with this explosion of knowledge on proteins and their interaction networks comes the potential to determine the fundamental principles underlying how biological activity is determined by protein structure. Aside from the academic value of structural and mechanistic studies on proteins and protein function, learning about the diversity of protein mechanisms also has practical outcomes. Proteins have evolved over many millions of years into structures primed for specific recognition and catalytic and regulatory activities. These inherent properties of proteins can be used to develop new technologies, such as nanomachines. Hence, we are witnessing a time that sees the union of science, medicine, and engineering for the advancement of biological mechanism and technological applications. Given the universality of protein recognition as both a mechanistic driving force in biology and disease and a functional driving force in biotechnology, one overarching challenge is to derive methods to identify and mechanistically characterize protein interactions and consequent protein recognition networks.
During the past decade, biosensors have emerged as an important tool for the measurement of protein interactions. At the same time, interfacing biosensors and other types of affinity capture with the developing tools of mass spectrometry are leading to even greater potential for the high-resolution identification of proteins and their interactions. In this chapter, we describe recent developments and protocols for biosensor utilization, including the emerging application of biosensors interfaced with mass spectrometry. Section 1 outlines three methods devised by our group to dissect the molecular events involved in the initial stages of human immunodeficiency virus type 1 (HIV-1) infection. In addition, Section 1 describes the use of the biosensor to investigate the mode of cytokine recognition by the interleukin-5 receptor α-chain. Section 2 presents some recent studies designed to advance the application of current biosensors. In one effort, the utility of biosensors is being advanced by designing adaptable peptide interfaces, which will allow the use of one affinity surface for the characterization of multiple targets. In a second effort, ways are being investigated to broaden the scope of the interactions available for investigation using the biosensor, from soluble interaction partners to those that depend on hydrophobic elements, such as the lipid plasma membrane. These two areas are exemplified using, respectively, the coiled-coil system for molecular interfaces and the reconstitution of an integral membrane receptor in a lipid bilayer environment on the biosensor surface. Finally, Section 3 discusses the very promising union of surface plasmon resonance biosensors with mass spectrometry, and a protocol is provided that outlines the use of these two techniques in tandem to analyze the C-reactive protein (CRP) content of human plasma samples.
SECTION 1: CURRENT BIOSENSOR USE FOR CHARACTERIZING RECEPTOR MACHINES
In general, biosensors use a suitable sensor platform functionalized by a recognition interface—be it antibody, peptide, receptor, or ligand—that acts as a conduit to enable transduction of an interaction with a soluble complement into a detectable signal. Surface plasmon resonance biosensors, such as the commercially available BIAcore systems (see Chapter 19 of the second edition), detect changes in mass at the surface of a sensor chip, by recording the variations of the critical angle needed to produce total internal reflection (TIR) of a light beam. The critical angle and the corresponding TIR of the light excite surface plasmons in a thin metal layer (usually gold) present at the sensor surface of the chip in an angle-dependent manner. The gold surface on the surface plasmon resonance (SPR) sensor chip is usually covered with a self-assembled monolayer of alkyl thiols. This suppresses nonspecific binding and creates surfaces suitable for further chemical conversion in order to attach functional groups (Johnsson et al. 1991). Most of the commercially available sensor chips have a matrix of carboxymethylated dextran covalently attached (1–3 ng mm–2) that forms a flexible hydrogel of an estimated thickness of about 100 nm, depending on the type of chip used. This dextran matrix can be derivatized to incorporate a number of different functional groups and to allow for a variety of immobilization chemistries involving –NH2, –SH, carbohydrate, and carboxyl groups on the ligand to be immobilized. The excitation of plasmons at the surface by the critical angle and the TIR of the light occurs when the momentum of the light matches that of the plasmons. The momentum of the plasmons, and hence the incident angle, is dependent on the refractive index at the sensor surface. Over a certain refractive index increment, the change in the critical angle is proportional to the mass bound and is expressed as the arbitrary resonance units (RUs), whereby 1 RU equates to 1 pg mm–2. The change in RUs, expressed as a function of time, is then plotted graphically and displayed as a sensorgram.
Until now, SPR optical biosensors have been the predominant type of sensor used in the study of macromolecular interactions, and they represent a unifying technology for detecting interactions across a wide range of affinity and size. Optical biosensors record the association and dissociation of macromolecular complexes in real time, therein offering the opportunity to measure both equilibrium affinity constants and the on- and off-rate constants for biomolecular interactions. In this way, biosensors can provide information about the binding reaction that is lost with techniques that measure binding only at steady-state conditions, such as calorimetry. Detecting differences in on- and off-rates is important for mapping recognition events and dynamics in biological systems and for providing improved signatures to identify specific proteins or other macromolecules for disease diagnosis, environmental science, and bioweapon monitoring. We have integrated this methodology into diverse aspects of our studies of the CD4/HIV-1 envelope and interleukin-5/interleukin-5 receptor systems (Canziani et al. 1999; Chaiken 2001; Chaiken et al. 2001). We present protocols and results for these systems below.
SECTION 1A: USE OF AN SPR BIOSENSOR TO DISSECT MOLECULAR EVENTS INVOLVED IN HIV-1 INFECTION
The application of SPR-based optical biosensors has contributed extensively to understanding many of the functional aspects of HIV-1. SPR biosensors allow the analysis of real-time interactions of any biomolecule—be it protein, nucleic acid, lipid, carbohydrate, or small molecule—without the need for modification of molecules of interest. As such, the technology has been used to analyze the molecular interactions associated with virtually every aspect of the viral life cycle, including docking, replication, budding, and maturation. In addition, applied research, related to vaccine and inhibitory drug development, has benefited from SPR-based methods.
Studies in this group have focused on the use of biosensor technology to dissect the molecular events that precede the invasion of HIV-1 into the cell, events that are fundamentally reliant upon interactions of the viral envelope protein with cell-surface receptors. The viral membrane-associated Env protein is composed of a gp41 transmembrane trimer and three noncovalently associated gp120 surface glycoproteins. Viral infection is initiated by the interaction of gp120 with the extracellular portion of CD4 on the target cell. The binding of these two proteins promotes a conformational change in gp120 that increases its affinity for a cell-surface coreceptor, typically CCR5 or CXCR4 (Sattentau and Moore 1991; Sattentau et al. 1993; Trkola et al. 1996). This second binding event leads to further conformational changes that culminate in fusion of the viral and target cell membranes (Fig. 1) (Clapham and McKnight 2002).
Because of the pivotal role gp120 has in the infection process, blocking its interactions with cell-surface receptors has emerged as an attractive goal for preventing HIV infection. The HIV-1 Env proteins, however, exhibit unusual features that complicate their viability as targets, with the main complication being the apparent conformational flexibility of gp120 (Kwong et al. 2002; Xiang et al. 2002).
SPR-based assays have been developed that permit the direct quantification of important interactions involving protein components involved in the infection process (Fig. 2). Methods to quantitatively and qualitatively probe gp120:CD4 and gp120:coreceptor-surrogate monoclonal antibody (mAb) 17b interactions have been determined (Brigham-Burke et al. 1992; Zhang et al. 1999; Myszka et al. 2000). mAb 17b was isolated from an HIV-1-isolated individual and is a member of a class of anti-gp120 antibodies referred to as CD4-induced (CD4i), as mutational studies have shown that it binds in a conformationally dependent manner to an epitope that overlaps the coreceptor-binding site (Thali et al. 1993). The coreceptor-binding site, and also the 17b-binding epitope, is only fully exposed upon interaction of gp120 with CD4, and 17b binding by gp120 is also induced by addition of CD4. SPR assays that allow the monitoring of this synergistic effect of soluble CD4 (sCD4) upon gp120’s mAb 17b binding have also been standardized (Zhang et al. 1999). These two- or three-component assays provide a platform for the screening and characterization of potential entry inhibitors.
In the following section, we provide three SPR-based protocols designed to specifically examine key binding events of gp120: direct binding of gp120 to mAb 17b, the synergistic effect of 17b binding to gp120 induced by the addition of soluble CD4, and the interaction of gp120 with a molecule that mimics CD4 (a CD4 mimetic). In addition, a protocol is also provided that demonstrates how these interactions are open to disruption.
Application of SPR to gp120 Antagonist Screening
Despite the complexity of the initial stages of viral infection, several studies have indicated that the interaction of gp120, or the Env heterotrimer, with both its cognate and artificial ligands is open to disruption. Agents shown to disrupt interactions of gp120 with CD4 and the coreceptors CCR5/CXCR4 include the scyllatoxin-scaffolded CD4 peptidomimetics ([20AGSF23]-ST and its derivatives; Dowd et al. 2002); several glycopeptide antibiotics (Balzarini et al. 2003); small-molecule anti-HIV compound BMS-806 (Si et al. 2004), and its derivatives; and the phage-derived linear peptide 12p1 (Ferrer and Harrison 1999).
There are a number of ways in which an antagonist of gp120/Env interaction could potentially work, and these fall into two broad categories: (1) competitive and (2) noncompetitive. Competitive inhibitors of gp120 bind in a manner similar to that of a natural ligand of gp120/Env, such as CD4, and physically block a shared binding site. This form of inhibition is seen with the [20AGSF23]-ST mimetics, which compete for sCD4 binding (Dowd et al. 2002). However, an unfortunate side effect of CD4 mimicry is the enhancement of coreceptor-binding efficiency, an effect that would ultimately facilitate, not hinder, the infection process. Noncompetitive inhibitors of gp120, however, apparently make use of the plasticity of gp120/Env, binding to the protein and stopping the transition of the protein to a conformation that is ligand-binding or fusion-competent. 12p1 and also BMS-806 appear to work in an allosteric fashion, with 12p1 inhibiting functional interaction (Biorn et al. 2004) and BMS-806 inhibiting gp41 fusion (Si et al. 2004). Noncompetitive inhibitors, such as 12p1, with their potential for dual inhibition of CD4 and coreceptor binding, in addition to inhibition of viral infection, may prove useful as therapeutic leads for the treatment of HIV-infected individuals.
SPR-based competition protocols offer the unique opportunity to determine which rate process (association or dissociation) a particular inhibitor compound affects. With regard to HIV-1 and the screening of entry inhibitors, the ability to directly immobilize either CD4 or the coreceptor surrogate monoclonal antibody on a sensor surface and then observe their interactions with gp120 offers the opportunity to screen inhibitors that affect different steps in the entry process, i.e., the initial interaction with CD4 or the prefusion interaction with the coreceptor molecule. The following protocols first outline how to perform basic analyses of interactions between gp120 and the mAb 17, or CD4/CD4-like molecules. This is then followed by a protocol that combines elements of the preceding ones, to functionally characterize a known inhibitor of gp120—a scyllatoxin-scaffolded CD4 mimetic “miniprotein.”
Protocol 1.1: Three-component Binding Assay: The Enhancing Effect of sCD4 on gp120-17b Interaction
This protocol demonstrates the synergistic effect that binding of sCD4 to HIV-1 gp120 has on its subsequent interaction with mAb 17b. Experimental conditions are a modification of those from Zhang et al. (1999). Briefly, two affinity surfaces are created on a sensor chip: the specific interaction to be investigated (mAb 17b) and a reference surface (an irrelevant protein, in this case the mAb 2B6R). Either gp120 alone or gp120-CD4 complexes are passed over these surfaces, and the responses are recorded. The response from the reference surface is then subtracted from the response from the experimental 17b surface to obtain the specific binding response of gp120/gp120-CD4 to 17b. In this experiment, the addition of CD4 increases the affinity of gp120 for mAb 17b. This is manifested as a marked increase (22–30-fold) in the association rate of the interaction (Fig. 3).
MATERIALS
Caution: See Appendix for appropriate handling of materials marked with <!>.
Buffers and Reagents
Phosphate-buffered saline (PBS; Roche Diagnostics, Inc.). Make fresh.
100 mm Sodium citrate (pH 3.4) <!>
35 mm NaOH/1 m NaCl solution <!>. Make fresh.
Penicillin/streptomycin (Gibco) <!>. Store at –20°C.
Pluronic acid (Gibco) <!>. Store at room temperature.
Hygromycin (Gibco) <!>. Store at 4°C.
Hybridoma SFM media (Gibco). Store at 4°C away from light.
RPMI 1640 medium (Gibco). Stored at 4°C away from light.
1% Antibiotic/antimycotic (Gibco). Store at –20°C.
100 mm Copper sulfatel. Make fresh.
Drosophila S2 Media (Gibco). Store at 4°C away from light.
Fetal calf serum (FCS; Mediatech). Store heat-inactivated aliquots at –20°C.
10 mm HCl <!>. Make fresh.
Polysurfactant P20 (BIAcore AB). Store at 4°C.
10 mm Sodium acetate (pH 5.5) (BIAcore AB) <!>. Store at 4°C.
400 mm N-Ethyl-N′-(dimethylaminopropyl)carbodiimide (EDC; BIAcore AB) <!>. Store in 100-μl aliquots at –80°C.
100 mm N-Hydroxysuccinimide (NHS; BIAcore AB) <!>. Store in 100-μl aliquots at –80°C.
1 m Ethanolamine (pH 8.0) (BIAcore AB) <!>. Store in 100-μl aliquots at 4°C.
Cell Types
Stably transfected Drosophila Schneider 2 cells that express a specific isolate of gp120 from a metal-inducible promoter (Culp et al. 1991) were used. There are many variants of HIV-1, each with a slightly different primary sequence, conformation, and glycosylation: Each variant binds to CD4 and mAb 17b to a greater or lesser extent. In this particular protocol, we use the monomeric gp120 derived from HIV-1JRFL (Koyanagi et al. 1987). Antibody prepared from human hybridoma cells expressing mAb 2B6R, an antibody raised to the interleukin-5 receptor α-chain, is used as the control.
Proteins
Soluble Human T-cell receptor CD4 (Immunodiagnostics, Inc., MA)
Purified mAb 17b (Strategic Biosolutions, Newark, DE)
-
Purified mAb 2B6R
This is a control protein used to determine the amount of nonspecific binding. However, any other irrelevant protein could be used as a control.
Purified HIV-1JRFL gp120
Special Equipment
-
mAb F105-linked fast-flow Sepharose column
Prepare using NHS-activated Sepharose Fast Flow Sepharose (Amersham Biosciences) as per manufacturer’s instructions.
-
Sensor chip CM5 (BIAcore AB)
This sensor chip is coated with a 100-nm layer of carboxymethyldextran surface to allow immobilization of proteins of interest via different chemistries (Lofas and Johnnson 1990).
BIA3000 SPR Optical Biosensor (BIAcore AB)
Liquid chromatography system (such as FPLC, Amersham)
FiberCell Hollow Fiber Bioreactor (FiberCell Systems, Inc.)
Affi-Prep protein A analytical cartridge (Bio-Rad)
METHODS
Production and Purification of gp120
Detailed descriptions of the growth and maintenance of eukaryotic cell culture are beyond the scope of this chapter, but they can be found in Doyle et al. (1998). Briefly, cells are grown in Schneider Drosophila medium supplemented with 10% (v/v) heat-inactivated fetal calf serum (heat-inactivated by incubating for 1 hour at 57°C), 0.5% penicillin/streptomycin, 0.05% pluronic acid, and 300 μg ml–1 hygromycin. Cells are maintained until they reach a density of 4 × 106 ml–1 and then induced by addition of copper sulfate to a final concentration of 500 μm. The cells producing the gp120 have been engineered such that they secrete the gp120 protein into the cell media. Therefore, 6 days after induction, the cell-culture supernatant is harvested, filter-sterilized, buffer-exchanged (into 50 mm sodium phosphate at pH 7.4), and concentrated to a final volume of 300 ml. Purification of gp120 is carried out by passage through an F105 antibody affinity column and elution with 100 mm citrate at pH 3.4 (Culp et al. 1991). The purified protein is dialyzed into 1× PBS and stored at –20°C.
Production and Purification of 2B6R
Detailed descriptions of growth and maintenance of hybridoma cell lines are beyond the scope of this chapter, but they can be found in Doyle et al. (1998). Briefly, the hybridoma cell line producing 2B6R is maintained in RPMI 1640 medium (GIBCO) supplemented with 10% (v/v) heat-inactivated FCS. The cells producing 2B6R have been engineered such that they secrete 2B6R mAb constitutively into the cell media. Cells are expanded and maintained until 500 ml of cell-culture supernatant can be harvested. The monoclonal antibody is then purified from this cell-free supernatant by FPLC using an Affi-Prep protein A analytical cartridge (Bio-Rad) as per manufacturers recommended protocols. Purified 2B6R is then dialyzed into 1× PBS and stored at –20°C.
Immobilization of Monoclonal Antibodies to CM5 Biosensor Chip
The procedure below outlines a standard method for covalently attaching proteins and peptides to the carboxymethyldextran surface of sensor chips. This method utilizes the formation of NHS esters from a fraction of the carboxyl groups of the carboxymethyldextran matrix via reaction with NHS and EDC hydrochloride in H2O. The protein to be immobilized is passed over this activated surface in a solution of low ionic strength with a pH value below the isoelectric point of the protein. This causes the electrostatic preconcentration of the protein at the matrix due to attraction between the positively charged protein and the negatively charged dextran. This step increases the availability and the probability of reaction of the free amine groups on the protein with the active esters on the matrix. Finally, any remaining active esters are converted into unreactive amides via reaction with ethanolamine.
This immobilization strategy results in the formation of a heterogeneous surface whereby the protein or ligand is attached at different sites. For some ligands, this approach may not be the optimum. Therefore, other site-specific orientation chemistries do exist that allow the attachment of the ligand at a defined site and result in a homogeneous surface population (see Section 2).
Dock CM5 chip and prime the system a minimum of three times with PBS supplemented with 0.005% P20. Start a sensorgram, over flow cell 1 only, at a flow rate of 5 μl min–1.
Dilute mAb 2B6R to be immobilized to a final concentration of 20 μg ml–1 in 10 mm sodium acetate buffer (pH 5.0).
Mix equal volumes (50 μl) of EDC and NHS stock solutions to a final concentration of 200 mm EDC/50 mm NHS.
Inject 35 μl of EDC/NHS solution to activate the sensor chip surface. Manually inject monoclonal antibody solution across sensor surface until a ligand density of between 800 and 1200 RUs is achieved.
Inject 40 μl of ethanolamine to block any sites that have not reacted with protein.
Repeat Steps 2–5, however, this time using a mAb 17b solution and flow cell 2.
Biosensor-binding Assays
Direct Binding of gp120 to mAb 17b (Control Experiment to Define the Interaction in the Absence of CD4)
Binding experiments are performed at 25°C with PBS + 0.005% P20 as the running buffer and at a flow rate of 30 μl min–1. Concentrations in the range of 19–250 nm of gp120 diluted in PBS + 0.005% P20 are injected simultaneously across both the mAb 2B6R and 17b surfaces for between 2 and 4 minutes. Between injections of analyte, it is desirable to remove any bound gp120 and regenerate the surface before proceeding with the next experiment. To do this, a regeneration buffer that is stringent enough to strip the analyte off the ligand without denaturing the ligand must be chosen empirically. For this interaction, removal of any remaining bound gp120 from 17b is achieved by pulses of 10 mm HCl at a flow rate of 100 μl min–1, until the baseline returns to the value before exposure to gp120 analyte.
Enhanced Binding of gp120 to mAb 17b in the Presence of Soluble CD4
Binding experiments are performed at 25°C with PBS + 0.005% P20 as the running buffer and at a flow rate of 60 μl min–1. The flow rate of a particular experiment is determined by the association rate of the interaction. Therefore, in the presence of CD4, the flow rate must be increased, as the gp120-17b interaction is augmented. In addition, the synergistic effect of CD4 on the gp120-17b interaction allows the use of lower initial concentrations of gp120. Therefore, concentrations in the range 2.5–125 nm of gp120 diluted in PBS + 0.005% P20 are mixed with a 15-fold excess of soluble CD4 (sCD4) at room temperature at least 1 hr prior to injection. The monoclonal antibody surfaces are regenerated using 10 mm HCl, as before.
SPR Data Analysis
To obtain sensorgrams that represent the specific binding of gp120 or gp120-CD4 to immobilized mAb 17b, the response from the reference cell (containing the interleukin-5 receptor α-specific mAb 2B6R) and the response from buffer alone are subtracted (termed “double referencing” [Myszka 1999]). After double-reference subtraction, the sensorgrams can be analyzed using BIAevaluation software to obtain kinetic constants.
Protocol 1.2: Characterization of Scyllatoxin-scaffolded CD4 Mimetics
This protocol allows multiple effects of miniprotein CD4 mimetics to be investigated using one biosensor chip derivatized with different proteins. The scyllatoxin-scaffolded CD4 mimetic miniproteins, such as [20AGSF23]-ST have three main binding characteristics: (1) They directly bind to gp120, (2) this binding to gp120 competes for gp120’s binding to sCD4, and (3) binding of the mimetic to gp120 will enhance the binding of gp120 to mAb 17b. Direct binding to gp120 is achieved by immobilization of HIV-1YU2 gp120 (or another gp120 variant) to one flow cell, CD4 competition via a second CD4-derivatized flow cell, and enhancement of coreceptor binding by immobilization of mAb 17b to a third flow cell (Fig. 2). As with all biosensor assays, a reference flow cell is created by immobilization of a nonspecific protein. In this case, this is the interleukin-5 receptor α-specific mAb 2B6R, applied to a fourth flow chamber. Experimental conditions and results are a modification of those from Dowd et al. (2002).
MATERIALS
Buffers and Reagents
Refer to Protocol 1.1
Cell Types
Same as those in Protocol 1.1, except for stably transfected Drosophila Schneider 2 cells expressing HIV-1YU2 gp120 (Li et al. 1991) from a metal-inducible promoter (Culp et al. 1991).
Proteins
Refer to Protocol 1.1
Scyllatoxin CD4 miniprotein mimetics (synthesized by standard solid-phase peptide chemistry as outlined in Dowd et al. 2002)
Special Equipment
Refer to Protocol 1.1
METHODS
Immobilization of Proteins to CM5 Biosensor Chip
Immobilization of each of the protein ligands of interest is accomplished using the standard amine coupling procedure outlined in Protocol 1.1. Proteins (and miniprotein depending on the particular orientation the experiment is to be carried out; see below) are diluted to the following concentration in coupling buffer (10 mm sodium acetate at pH 5.5): mAb 2B6R to 20 μg ml–1, gp120 to 84 μg ml–1, or miniprotein to approximately 1 μg ml–1; CD4 to 55 μg ml–1; and mAb 17b to 20 μg ml–1. Each different protein is immobilized on a separate flow channel.
Biosensor-binding Assays
(A) Direct Binding of CD4 Mimetics to gp120
Direct-binding assays can be performed in either of two ways: (1) with gp120 immobilized on the chip surface (as in Fig. 2a2) or (2) with the miniprotein immobilized (as in Fig. 2a1). Binding experiments are performed at 25°C with PBS + 0.005% P20 as the running buffer. Below is a description of the second option, with the [20AGSF23]-ST CD4 mimetic immobilized on the sensor surface. As SPR-based biosensors primarily detect changes in mass at the sensor surface (refer to introduction to Section 1), due to the large size of the gp120 analyte, a relatively small amount of the CD4 miniprotein can be attached to the sensor surface. Typically, 50–400 RUs will give a sufficient signal from which to obtain kinetic data. Binding experiments are performed at 25°C with PBS + 0.005% P20 as the running buffer and at a flow rate of 10 μl min–1. HIV-1YU2 gp120 in the concentration range 6.25–300 nm diluted in PBS + 0.005% P20 is injected across the miniprotein surface for a 5-minute association and 2-minute dissociation. Removal of any remaining bound gp120 is achieved by pulses of 35 mm NaOH/1.3 m NaCl at a flow rate of 100 μl min–1 until the baseline returns to the value before exposure to gp120 analyte. Representative data obtained by this method are shown in Figure 4A.
(B) CD4 Competition
Competition for CD4 binding by the miniprotein mimetics is achieved by titration of the miniprotein into the interaction of gp120 with immobilized soluble CD4 (sCD4). CD4 is bound to the surface at a density of between 900 and 1500 RUs. Binding experiments are performed at 25°C with PBS + 0.005% P20 as the running buffer. HIV-1YU2 gp120 (100 nm) and solutions of peptide (39.5–200 μm) mixed with gp120 (100 nm) are prepared in PBS + P20 and passed over the sensor surface at a flow rate of 70 μl min–1 for 1 minute. Regeneration of the surface between injections is described as above in Protocol 1.2A. Representative data obtained by this method are shown in Figure 4B.
(C) Enhanced Binding of gp120 to mAb 17b in the Presence of CD4 Mimetics
Binding experiments are performed at 25°C with PBS + 0.005% P20 as the running buffer. mAb 17b is immobilized to a final surface density of between 600 and 1200 RU. HIV-1YU2 gp120 (100 nm) and solutions of peptide (0.025–330 μm) plus gp120 (100 nm) are prepared in PBS + P20 and passed over the sensor surface at a flow rate of 70 μl min–1 for 1 minute. The surfaces are regenerated by one 20-μl injection of 10 mm HCl at a flow rate of 100 μl min–1. Representative data obtained by this method are shown in Figure 4C.
SPR Data Analysis
To obtain sensorgrams that represent the specific binding of the gp120-miniprotein interaction or the gp120:miniprotein-mAb 17b interaction, a double-reference subtraction is performed as outlined in Protocol 1.1, after which the sensorgrams can be analyzed using BIAevaluation software to obtain kinetic constants.
Protocol 1.3: Characterization of Inhibitors of HIV-1 gp120 Interactions
As with Protocol 1.2, this protocol makes use of one biosensor chip derivatized with different proteins. However, in this instance, these surfaces are employed to observe the different properties of a particular inhibitor. Direct binding of the inhibitor to HIV-1 gp120, the effect of the inhibitor upon the gp120-CD4 interactions, and its effect upon gp120-mAb 17b binding can all be investigated. Although this protocol is applicable to virtually all soluble gp120 inhibitors, the example given is the linear peptide inhibitor 12p1. The linear peptide 12p1 (RINNIPWSEAMM) was previously isolated from a phage display library and was found to inhibit interaction of HIV-1 gp120 from a number of different strains, with both CD4 and a CCR5 surrogate, mAb 17b (Ferrer and Harrison 1999). Experimental conditions and results are taken from Biorn et al. (2004).
MATERIALS
Buffers and Reagents, Cell Types, Proteins, Special Equipment
Refer to Protocol 1.1
12p1 linear peptide (synthesized by standard solid-phase peptide chemistry as outlined in Biorn et al. 2004).
METHODS
Immobilization of Proteins to CM5 Biosensor Chip
Refer to Protocol 1.2
Biosensor-binding Assays
Direct Binding of 12p to gp120
Direct-binding assays are performed with HIV-1YU2 gp120 immobilized on the chip surface (2000 RUs) at 25°C with PBS + 0.005% P20 as the running buffer and a flow rate of 5 μl min–1. Different concentrations of 12p1 peptide (1–562 μm) are passed across the gp120 surface for a 5-minute association phase and 2-minute dissociation phase. Due to the relatively weak nature of the 12p1 interaction, a regeneration step is not required. 12p1 directly binds to gp120, with a 1:1 stoichiometry; therefore, as the concentration of 12p1 increases the response, so should the SPR response (shown in Fig. 5A).
Effect of 12p1 on the gp120-CD4 Interaction
To observe the effects that the 12p1 peptide has upon the interaction of HIV-1YU2 gp120 with CD4, gp120 with increasing concentrations of 12p1 are passed over a CD4 surface (800–1000 RUs). HIV-1YU2 gp120 (50 nm) in the absence (0 nm) or presence of 12p1 (0.821–6.57 μm) is passed over the CD4 surface, and the response is recorded. Binding experiments are performed at 25°C with PBS + 0.005% P20 as the running buffer. Removal of any remaining bound gp120 is achieved by pulses of 35 mm NaOH/1.3 m NaCl at a flow rate of 100 μl min–1 until the baseline returns to the value before exposure to gp120 analyte. Because 12p1 is an inhibitor of the gp120-CD4 interaction, as the concentration of 12p1 increases, the response from the gp120-CD4 interaction decreases (shown in Fig. 5B).
Effect of 12p1 on the Binding of gp120 to mAb 17b
To observe the effects that the 12p1 peptide has on the interaction of gp120 with mAb 17b, gp120 with increasing concentrations of 12p1 are passed over a mAb 17b surface (800–1000 RUs). HIV-1YU2 gp120 (50 nm) in the absence (0 nm) or presence of 12p1 (0.821–6.57 μm) is passed over the 17b surface, and the response is recorded. Binding experiments are performed at 25°C with PBS + 0.005% P20 as the running buffer. Removal of any remaining bound gp120 is achieved by pulses of 10 mm HCl at a flow rate of 100 μl min–1 until the baseline returns to the value before exposure to gp120 analyte. 12p1 is an inhibitor of the gp120-17b interaction; therefore, as the concentration of 12p1 increases, the response from the gp120-17b interaction decreases (shown in Fig. 5B).
Saturation Curve Analysis: Determining the Mode of Action of the 12p1 Inhibitor
If an inhibitor works by competing with a given ligand, and the affinity of this ligand for the particular target is greater than the affinity of the inhibitor for the target, at a certain concentration of ligand, the effect of the inhibitor should be negligible. However, if the mode of action of the inhibitor is noncompetitive or allosteric in nature, no amount of ligand will be able to negate the effect of the inhibitor. This mode of action of an inhibitor can be investigated using an SPR saturation curve analysis in the absence or presence of the inhibitor. If the inhibitor is competitive, at a certain concentration of ligand, the response in the presence of inhibitor will reach the maximum binding capacity of the interaction in the absence of inhibitor. However, if the mode of action of the inhibitor is allosteric, this maximum binding response will never be achieved.
To ascertain the mechanism, competitive or noncompetitive, by which 12p1 exerts its inhibitory effect, CD4 saturation analyses in the presence or absence of 12p1 are performed. An HIV-1YU2 gp120 surface (2400 RUs) is challenged with increasing sCD4 concentrations (0–3.5 μm) in the presence of 100 μm 12p1 at a flow rate of 5 μl min–1. Solutions are injected for a 5-minute association phase, followed by a 5-minute dissociation phase. HIV-1YU2 gp120 surfaces are regenerated with 35 mm NaOH and 1.3 m NaCl. As 12p1 acts in an allosteric fashion to inhibit the key interactions of gp120, the maximum response of the interaction in the presence of 12p1 never reaches the maximum response in the absence of 12p1 (shown in Fig. 5C).
SPR Data Analysis
To obtain sensorgrams that represent the specific binding of the gp120-CD4 interaction or the gp120-mAb 17b interaction, in the presence or absence of peptide, a double-reference subtraction is performed as outlined in Protocol 1.1, after which the sensorgrams can be analyzed using BIAevaluation software to obtain kinetic constants.
SECTION 1B: “SANDWICH” SPR ASSAY
The use of SPR reveals unique binding and recognition modes in cytokine-receptor assembly. The techniques described above require purified protein made in an overproduction system. The following protocol describes a sandwich SPR biosensor method, in which transiently expressed proteins are selectively captured from heterogeneous cell-culture media by an antibody, providing a homogeneous surface for the subsequent quantitative kinetic binding assay. Figure 6 depicts a sandwich binding assay that uses a carboxy-terminal V5 tag and anti-V5 antibody (Ishino 2004). The use of the V5 tag system has multiple advantages, not the least of which is the ability to orient the receptor towards the solvent, mimicking the natural orientation of the receptor on the cell surface. Additionally, mutations within the receptor do not affect the capturing, since it is mediated by an exogenous sequence grafted onto the carboxyl terminus. We have successfully utilized this protocol to investigate the kinetics of interaction of 25 mutational variants of interleukin-5 receptor α (IL5Rα). In addition, we have also used a similar antibody capture procedure to measure binding of the IL5Rα to antibody-anchored variants of IL5) (Fig. 6ii) (Morton et al. 1995). The basics of these two methods are outlined in Figure 6, and the relevant biology is summarized below.
Biology
The activation of cytokine receptors is a stepwise process that depends on (1) their specific extracellular interaction with cognate cytokines, (2) the formation of oligomeric receptor complexes, and (3) the subsequent initiation of cytoplasmic phosphorylation events that elicit complex cellular responses. These steps then culminate in the elicitation of a cellular response. SPR biosensors can provide a powerful means to analyze the initial cytokine-soluble receptor interactions, as well as the formation of important oligomeric multiprotein assemblies, allowing the extraction of kinetic information and providing insight into potential mechanistic models of the activation process. This information eventually assists in the design of cytokine-receptor antagonists, to correct aberrant physiological responses. Studies within our group have utilized the SPR biosensors in exactly this manner to study the molecular recognition mechanisms of human IL5 and its receptor α.
IL5 is a T-cell-derived cytokine that has a central role in the differentiation and activation of eosinophils (Karlen et al. 1998). Since eosinophils cause inflammatory diseases such as asthma and hypereosinophilic syndrome, an IL5 antagonist would be expected to be a useful pharmaceutical agent (Foster et al. 2002). Initial IL5 binding to IL5Rα on the eosinophil cell surface forms a complex that then recruits a common β receptor (βc) to induce cytoplasmic signal transduction (Fig. 7). Studies have shown that human IL5Rα expressed alone in cells binds IL5 with an equilibrium dissociation constant (KD) of 0.3–0.6 nm (Murata et al. 1992). This binding affinity is increased only two- to fivefold when the IL5Rα and β chains are coexpressed (Tavernier et al. 1991). In other words, IL5Rα provides most of the IL5-binding energy, whereas βc is essentially for signaling. Like most cytokine receptors, IL5Rα consists of an extracellular region, a single transmembrane region, and a cytoplasmic region. Finally, the SPR protocol below (and other detailed biophysical studies of the chimokine receptor interaction) has been facilitated by the generation of a soluble form of IL5Rα (sIL5Rα) that contains only the extracellular region (Tavernier et al. 1992).
Protocol 1.4: Sandwich-binding Assay Using Anti-V5 Tag Antibody: Combination of Alanine-scanning Mutagenesis with Kinetic Analysis to Investigate IL5 Recognition by IL5Rα
Below is a detailed description of a combinatorial alanine-scanning mutagenesis:kinetic interaction analysis of IL5Rα (Figs. 8 and 9). This kind of analysis can be performed to define specific residues involved in ligand-receptor molecular recognition and was used in our group to define those residues in IL5Rα important for the recognition of IL5 (Fig. 9).
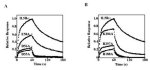
MATERIALS
Caution: See Appendix for appropriate handling of materials marked with <!>.
Buffers and Reagents
Refer to Protocol 1.1
10 mm Glycine-HCl (pH 1.5 and pH 2.0) (BIAcore AB) <!>
Drosophila Serum-free Media (Invitrogen)
Drosophila Complete Media (Invitrogen)
l-Glutamine solution (Invitrogen)
Cell Types
Proteins
Anti-human IL5Rα polyclonal antibody (R&D Systems)
Anti-histidine tag polyclonal antibody (Santa Cruz Biotechnology)
Anti-V5 tag monoclonal antibody (Invitrogen)
α16 (nonneutralizing anti human IL5Rα monoclonal antibody; Tavernier 2000)
-
IL5 protein
Prepare as described previously by Scibek et al. (2002).
mAb 17b
pMT-V5/His-sIL5Rα and derivatives
METHODS
Production of Human sIL5Rα and Its Mutants
Drosophila S2 cells are transfected with the metal-inducible vector pMT-V5/His expressing wild-type sIL5Rα and mutant derivatives produced by alanine scanning and grown in 3 ml of serum-free medium supplemented with 20 mm l-glutamine. Protein expression is induced by addition of copper sulfate to a final concentration of 600 μm 3 days after transfection. The cell-free supernatant is then collected after 2 days and stored at –20°C prior to the binding analysis described below. The level of receptor expression in cell-culture supernatants is measured by western blot using anti-human IL5Rα polyclonal antibody and anti-histidine tag polyclonal antibody. Protein concentration is usually about 200 nm, although different alanine scan mutants may be present at different (generally lower) levels.
Immobilization of Proteins to CM5 Biosensor Chip
Immobilization of antibodies to V5 or to sIL5Rα is carried out using the amine-coupling method described in Protocol 1.1.
Affinity Capture and On-chip Purification of IL5Rα
Cell-culture supernatants are passed across the two flow cells at a flow rate of 10 μl min–1. As cell-culture supernatants are used, and there are natural differences in expression between wild-type and alanine variants of the IL5Rα, the contact time for the affinity capture of individual proteins must be adjusted. The expressed sIL5Rα from cell supernatants is specifically captured by virtue of the antibodies to either the V5 tag or the sIL5Rα-specific antibodies immobilized on flow cells (Fig. 8A), whereas other components of the supernatants pass through the sensor chip flow cells without binding. Generally, the injection is continued until approximately 250 RUs of sIL5Rα or its derivatives are immobilized (Fig. 8B).
Kinetic-binding Assay
The kinetic interaction assay of IL5 binding to captured IL5Rα is performed using a BIAcore 3000 optical biosensor (BIAcore AB). All of the experiments are conducted at 25°C in PBS buffer with 0.005% P20 at a flow rate of 50 μl min–1. Concentrations of IL5 ranging from 0 to 50 nm are injected across all surfaces for a 1-minute association phase and a 2-minute dissociation phase (Fig. 8C). Between injections, the entire surface is regenerated by two 15-second pulses of 10 mm glycine (pH 1.5) (Fig. 8D).
SPR Data Analysis
To obtain sensorgrams that represent the specific binding of the IL5 to affinity-captured sIL5Rα, a double-reference subtraction is performed as outlined in Protocol 1.1, after which the sensorgrams can be analyzed using BIAevaluation software to obtain kinetic constants. Characteristic sensorgan differences for mutants that reduce the IL5/IL5R interaction affinity are shown in Figure 9.
SECTION 2: ADVANCING BIOSENSOR INTERFACES FOR MULTIPLEXED ARRAYS AND MEMBRANE ENVIRONMENT ANALYSES
Although there are clear benefits of biosensors for protein interaction analysis, there also are important limitations on current usage. One key limitation is that the current optical biosensors possess a limited range of robust molecular interface designs for incorporation of diverse binding specificities on the sensor surface, including for high-throughput arrays. Availability of more diverse specificities on sensor surfaces would improve the potential use of multiplexed biosensor analysis for investigation of the large “interaction space” of living systems (the “interactome”). A second limitation is that biosensors have been utilized mainly for soluble proteins or soluble domains of proteins (e.g., receptor ectodomains as in the previous example), whereas many biologically important interactions occur in membrane environments: These have largely been unavailable to date in biosensor interaction analysis. Newly evolving interface systems, in conjunction with developing sensor platform technologies, should greatly enhance biosensor technologies. The following examples and protocols highlight some emerging possibilities.
SECTION 2.1: COILED-COIL PEPTIDES AS ADAPTABLE MODULAR INTERFACES
One source of novel interfaces for next-generation biosensors may be intelligently designed “miniproteins.” Miniproteins are conformationally stable domains, small enough to be amenable to chemical synthesis, and they provide a native, protein-like conformational order useful for designing sensor interfaces with desired conformational and recognition activities. The coiled-coil domain (Fig. 10), like the leucine zipper, is an α-helical oligomer commonly found in many naturally occurring proteins (transcription factors such as GCN4, viral fusion peptides, certain tRNA synthetases, tropomyosin, etc.) and often used to control oligomerization (Banner et al. 1987; Cohen and Parry 1990; Cusack et al. 1990). The coiled-coil motif represents one of the simplest tertiary structures in proteins, involving a number of α-helices wound around each other in a highly organized manner. There can be between two and five helices in the coiled-coil structure, although dimers and trimers are most common. The structural stability of a coiled-coil is maintained largely by an interface created by the supercoiled packing of hydrophobic side chains from aligned constituent helices. This basic folding scheme has been confirmed by both nuclear magnetic resonance (NMR) and crystallographic analyses of the coiled-coil region of transcriptional activator GCN4 (O’Shea et al. 1991) and a coil-Ser peptide (Lovejoy et al. 1993). In contrast to single-stranded α-helical peptides, the coiled-coil is highly stable in aqueous solution (Hodges et al. 1990; Oas et al. 1990).
Most coiled-coil sequences contain heptad repeats: seven-residue patterns denoted abcdefg, in which the a and d residues (core positions) are generally hydrophobic. As there are 3.6 residues to each turn of the α-helix, these a and d residues form a hydrophobic seam, which, as each heptad is slightly under two turns, slowly twists around the helix. The coiled-coil is formed by component helices twisting around each other to bury their hydrophobic seams, thus forming a supercoil. It is the characteristic interdigitation of side chains between neighboring helices, known as knobs-into-holes packing, that defines the structure as a coiled-coil (Crick 1953). Residues in positions e and g are usually charged and contribute to electrostatic interaction between supercoiled peptides. A coiled-coil interaction can occur with antiparallel helices, although the parallel conformation is more common. An antiparallel conformation is rare in trimers and unknown in pentamers, but more common in intramolecular dimers, where the two helices are often connected by a short loop. Although the heptad sequence repeat arrangement is most prevalent in coiled-coils, alternative packing strategies also exist. Hendecad repeats (11 residues) are found in long filamentous proteins, and such alternative repeat patterns may contribute to partner choice (Hicks et al. 1997).
The coiled-coil unit embodies several key structural features that make it of great potential value in designing molecular interfaces for biosensor development. Perhaps the most important is that it contains two or more noncovalently assembled helical peptide building blocks. One of these can be attached covalently to a biosensor surface by coupling an incorporated cysteine through a ligand thiol procedure, whereas a second can associate noncovalently from solution. Because the second coil can be designed with variable binding epitopes, the coiled-coil unit can be assembled with adaptable binding functions at different sites on the sensor surface. The second coil can also be fused with a protein of particular interest, such as a receptor, to enable an oriented and specific immobilization on biosensor surfaces (De Crescenzo et al. 2003). Furthermore, the pattern of hydrophobic interface and charged border residues can be varied to produce coiled-coil trimers as well as dimers (Betz et al. 1995; Burkhard et al. 2002). Therefore, coiled-coils have great potential for providing function-adaptable biosensor interfaces. These can be used to develop sensors with arrays of specificities, which can be changed in situ by replacing the recognition module. Since coiled-coil formation from single-chain helices embodies significant conformational change, these molecular species also provide tools to evaluate the extent to which newly evolving platforms can detect conformational changes.
Protocol 2.1: Coiled-coiled Heterodimer Assembly Study and Antibody Epitope Recognition
This experiment shows the ability of two newly designed coiled-coil peptides, MSK2 and MSE3, to self-assemble on a BIAcore biosensor surface. MSK2 contains cysteine as final amino acid at the carboxyl terminus, allowing a specific and oriented immobilization on the chip surface (Fig. 11). MSE3, the analyte peptide, contains an epitope sequence, hexa-His, which can be recognized by monoclonal antibodies. The ability to graft protein recognition motifs on the analyte peptide or fuse the peptide to a larger protein will prove useful in the realization of a multiplexed screening system.
MATERIALS
Caution: See Appendix for appropriate handling of materials marked with <!>.
Buffers and Reagents
Refer to Protocol 1 for standard reagents
Side-chain-protected Fmoc-amino acids (Anaspec)
2-(1-H-benzotriazole-1-yl)-1,1,3,3-tetramethyluronium hexafluorophosphate (HBTU; NovaBiochem) <!>
N-Hydroxybenzotriazole · H2O (HBOt; NovaBiochem, San Diego, CA)
Penta-His monoclonal antibody (Qiagen)
2-(2-Pyridinyldithio)-ethaneamine hydrochloride (PDEA; BIAcore AB)
Cysteine hydrochloride (Sigma-Aldrich) <!>
N-Methyl-2-pyrrolidinone (NMP; Fischer Scientific) <!>
Dichloromethane (DCM; Fischer Scientific) <!>
Piperidine (Sigma-Aldrich) <!>
Methanol (Fischer Scientific) <!>
N,N-Diisopropylethylamine (DIEA; Fischer Scientific) <!>
Fmoc-PAL-PEG-PS resin (Applied Biosystems)
Trifluoroacetic acid (TFA; Fischer Scientific) <!>
Thioanisole (Sigma-Aldrich) <!>
1,2-Ethanedithiol (Sigma-Aldrich) <!>
Acetonitrile (Fischer Scientific) <!>
Special Equipment
METHODS
Peptide Synthesis and Purification
The MSK2 and MSE3 peptides are prepared by solid-phase synthesis methodology using conventional Fmoc (9-fluorenylmethoxycarbonyl) chemistry on an Applied Biosystems Model 433A peptide synthesizer, 0.1 mmoles scale, using Fmoc-PAL-PEG-PS resin. The MSK2 sequence is EKVSALKDKVSALKRKVSALKDKVSALKRKVSALKDGGC (39 amino acids); the MSE3 sequence is HHHHHHGGGEVSALEDEVSALEREVSALEDEVSALEREVS-ALEDK (45 amino acids). Peptides are cleaved from the resin by reaction with 95% TFA containing 2% (v/v) thioanisole and 4% (v/v) 1,2-ethanedithiol for 3 hours at room temperature. Crude peptides are then precipitated with cold diethyl ether and dried. Purification of each peptide is performed by reversed-phase high-performance liquid chromatography (RP-HPLC) on a Vydac semipreparative C18 column (250 × 10-mm inner diameter, 10-mm particle size, 300-Å pore size) with a linear AB gradient (ranging from 5% to 60% B in 40 minutes) at a flow rate of 5 ml/min, where solvent A is aqueous 0.1% (v/v) TFA in mQ H2O and solvent B is 0.1% TFA in acetonitrile. Homogeneity of the purified peptides is verified by analytical RP-HPLC, amino acid analysis, and MALDI mass spectrometry.
Immobilization of MSK2 Peptide on CM5 Biosensor Chip
Covalent coupling of the cysteine-containing MSK2 peptide to the biosensor CM5 chip surface is achieved using the ligand thiol method (Fig. 12) as described previously (Johnsson et al. 1991). This method allows the oriented immobilization of a protein or peptide on the sensor surface via a single cysteine residue.
Dock a CM5 chip, and prime the system a minimum of three times with PBS supplemented with 0.005% P20. Start a sensorgram over flow cell 1 only, at a flow rate of 5 μl min–1.
Dilute the MSK2 to be immobilized to a final concentration of 100 nm in 10 mm sodium acetate buffer (pH 4.0).
Mix equal volumes (50 μl) of EDC and NHS stock solutions to a final concentration of 200 mm EDC/50 mm NHS.
Inject 10 μl of EDC/NHS solution to activate the sensor chip surface, followed by 20 μl of 80 mm PDEA in 100 mm borate buffer (pH 8.5). Manually inject MSK2 solution across sensor surface until a ligand density of 200 RUs is achieved.
Inject of 20 μl of 50 mm cysteine, 1 m NaCl, 0.1 m formate (pH 4.3) to block the remaining activated sites.
Use the same procedure to immobilize MSK2 on flow cells 2 and 3 to obtain immobilization densities of 500 and 1000 RU, respectively. Different levels of immobilized MSK2 on different flow cells allow evaluation of the impact of the ligand density in the MSE3-binding experiment described below. Flow cell 4 serves as a reference surface. This surface is activated with EDC/NHS, followed by PDEA, but is then blocked immediately with 50 mm cysteine, 1 m NaCl, and 0.1 m formate (pH 4).
Biosensor-binding Assays
Direct Binding of MSE3 (Analyte Peptide) to MSK2 (Ligand Peptide)
All kinetic experiments are performed on a BIAcore BIA 3000 optical biosensor instrument at 25°C with PBS supplemented with 0.005% P20 as the running buffer. Different concentrations of MSE3, ranging from 2.5 to 50 nm, are injected across all chip flow cells for 90 seconds (association phase); dissociation phase is performed in 90 seconds at the same flow rate (50 μl min–1). Between runs, removal of bound MSE3 (surface regeneration) is achieved by two 60-second injections of 0.1% SDS at 50 μl min–1.
Recognition of Antibody Epitope on Captured MSE3 Peptide
In a three-component sandwich assay, it is possible to test the recognition of the 6 × His epitope by a Penta-His-directed monoclonal antibody. For this purpose, MSE3 is captured on the MSK2 surface by injecting MSE3 at 100 nm solution for 90 seconds. This will result in final density of 150 RU. Across this surface, solutions of different concentrations of antibody (5–100 nm) are injected with a 90-second association and 90-second dissociation periods.
SPR Data Analysis
Sensorgrams collected with different analyte concentrations (MSE3 or Penta-His monoclonal antibody) are pooled and analyzed to establish affinity and kinetic profits for the MSE3-MSK2 and Penta-His monoclonal antibody-MSE3 interactions. The response from a reference cell and the response from buffer alone are subtracted from each set of sensorgrams for “double referencing.” In the direct binding of MSE3 to MSK2, the reference surface is an empty flow cell, activated and subsequently deactivated; in the case of binding of Penta-His monoclonal antibody to captured MSE3, the reference is a flow cell containing MSK2. After double-reference subtraction, the sensorgrams can be analyzed using BIAevaluation software to obtain kinetic constants.
Data obtained for binding of synthesized coiled-coil peptides show that MSE3 interacts with immobilized MSK2 (Fig. 13A) with a dissociation constant in the nanomolar range (KD 2.04 nm), a kon of 3.00 × 105 m–1s–1, and a slow dissociation rate, koff 6.26 × 10–5 s–1. This last aspect supports the application of coiled-coil peptides for immobilization of biomolecules on biosensor surfaces, in an oriented fashion. Subsequent demonstration that the linear epitope sequence present at the amino terminus of MSE3 peptide, hexa-Hist, is able to be recognized by a specific monoclonal antibody (KD 48.92 nm, kon 2.52 × 105 m–1 s–1, koff 12.16 × 10–3 s–1), raises the possibility that this system can be used to develop an array analysis system (Fig. 13B).
SECTION 2.2: SENSING IN MEMBRANE ENVIRONMENTS
As SPR-based applications become more widespread, so the desire to expand the diversity and utility of optical biosensors has become increasingly compelling. To date, SPR-based optical biosensors have been used predominantly to characterize interactions that occur within an aqueous environment. However, efforts to develop methods to measure interactions that occur in a hydrophobic context, such as the cell membrane, have been mounted. A number of studies have monitored (1) the interaction of peptide/protein–membrane interactions (Hong et al. 2002), (2) the excitation of a membrane-embedded protein (Karlsson and Lofas 2002), and, most recently, (3) the interactions of proteins with a functional protein reconstituted in a membrane (Stenlund et al. 2003).
Functional Reconstitution of Integral Membrane Proteins within a Membrane Environment
Cellular receptors have critical roles in many physiological processes, including cell-to-cell signaling, modulation of cellular adhesion, and transduction of signals to the cell from an environmental stimulus. Of the numbers and various types of receptors on a cell, the broad family of G-protein-coupled receptors (GPCRs) represents a vast set of proteins that are well conserved in many prokaryotic and eukaryotic systems (Palczewski et al. 2000; Fredriksson et al. 2003). Karlsson and Lofas (2002) and, more recently, Stenlund et al. (2003) have carried out studies in which they successfully reconstituted a GPCR family member on a biosensor surface and monitored either the excitation of the immobilized protein by light or its interaction with protein ligands. Studies in our group have adapted and extended these published methods to investigate the influence of microdomain components, such as cholesterol, and phospholipid hydrocarbon chain length on the captured protein. These studies focused on the pathophysiologically relevant GPCR, CCR5 (involved in HIV-1 infection; see Fig. 1), a receptor that until recently was unavailable for studies with chemokines or conformationally sensitive antibodies.
The following protocol focuses on ongoing studies performed within our group designed to fully reconstitute CCR5 within a lipid environment on a biosensor surface. An overview of the scheme is illustrated in Figure 14.
Protocol 2.2: Reconstitution of CCR5 in a Lipid Environment
This protocol outlines a procedure that functionally reconstitutes the HIV-1 coreceptor molecule CCR5 within a model membrane on a sensor chip. Theoretically, this could be applied to any membrane protein; however, the specific lipid components for the chosen membrane protein must be optimized individually. When starting an investigation into reconstitution of a membrane protein in a lipid environment on a sensor surface, a good starting lipid would be 1-palmitoyl-2-oleoyl-sn-glycero-3-phosphocholine (POPC), as this is considered a standard lipid in membrane research.
MATERIALS
Caution: See Appendix for appropriate handling of materials marked with <!>.
Buffers and Reagents
Dulbecco’s Modified Eagle’s Medium (DMEM; GIBCO)
5 mm EDTA in phosphate-buffered saline (PBS)
G418 (Mediatech, Inc.)
100 mm (NH4)SO4, Tris-HCl (pH 7.5), 10% glycerol, 1% Cymal-5, or CHAPSO <!>
100 mm (NH4)SO4, Tris-HCl (pH 7.5), 10% glycerol, 1% Cymal-5, or CHAPSO, 100 mm MgCl2 <!>
100 mm Sodium acetate (pH 5.5) <!>
10 mm Sodium acetate (pH 4.0) <!>
5 mm Carbohydrazide in H2O
100 mm Cyanoborohydride (Sigma) in 100 mm sodium acetate (pH 4.0). <!> Make fresh.
50 mm Sodium periodate (Sigma) stock solution in 100 mm sodium acetate buffer (pH 5.5)
1,2-Dimyristoyl-sn-glycero-3-phosphocholine (DMP; Avanti Polar Lipids)
3-[(3-Cholamidopropyl)dimethylammonio]-2-hydroxy-1-propanesulfonate (CHAPSO; Sigma)
Cymal-5 (Anatrace)
Cholesterol (Sigma)
10 mm HCl <!>
10 mm HEPES (pH 7.4), 150 mm NaCl (HBS-N; BIAcore AB)
Octyl-β-glucoside (Sigma)
Cell Types
Proteins and Peptides
-
mAb 2D7 (Pharmingen)
This antibody recognizes extracellular loop 2 of CCR5.
Purified 2B6R
-
mAb 1D4 (University of British Colombia)
This antibody recognizes the rhodopsin-derived peptide TETSQVAPA.
-
mAb 45523.1 (R&D Systems)
This antibody recognizes multiple domains on CCR5.
-
mAb CTC8 (R&D Systems)
This antibody recognizes the amino terminus of CCR5.
-
Regulated on activation, normal T-cell-expressed and -secreted (RANTES; PeproTech Inc.)
This is a natural chemokine (chemotactic cytokine) ligand of CCR5 involved in function.
TETSQVAPA (synthesized by standard solid-phase chemistry)
Special Equipment
-
Sensor Chip Pioneer L1 (BIAcore AB)
This sensor chip is composed of a CM-dextran surface with lipophilic groups attached to facilitate the capture of liposomes.
Rotovapor R110 (Brinkmen)
150-mm Petri dishes
-
1D4-coated Sepharose beads
Prepare beads using the Affi-Gel Hz Immunoaffinity Kit (Bio-Rad).
NAP-5 desalting column (Amersham Biosciences)
METHODS
Expression and Overproduction of Human Chemokine Receptor CCR5
The human chemokine receptor CCR5 is synthesized and overproduced in canine thymocyte Cf2Th cells in the same manner as described previously (Mirzabekov et al. 1999). Briefly, a cell line (Cf2Th/synCCR5) stably expressing human CCR5 is grown in DMEM containing 10% (v/v) fetal calf serum (FCS) and 0.4 μg ml–1 of G418. Cf2Th/synCCR5 cells grown to full confluency in 150-mm dishes are harvested using 5 mm EDTA in PBS, washed in PBS, pelleted, and frozen until needed. The carboxyl terminus of the expressed CCR5 consists of an engineered glycine residue followed by the C9 nonapeptide TETSQVAPA. This sequence serves as a tag recognized for the 1D4 antibody (Hodges et al. 1988). CXCR4 is derived in a similar manner using Cf2Th/synCXCR4 cells and is similarly tagged (Babcock et al. 2001).
CCR5 Solubilization and Purification
A pellet of 1 × 107 Cf2/synCCR5 cells is lysed with 1 ml of a solubilization solution (100 mm (NH4)2SO4, 20 mm Tris-HCl [pH 7.5], 10% glycerol, 1% Cymal-5 or 1% CHAPSO). The cells are tumbled for 30 minutes, and the insoluble fraction is pelleted by centrifugation at 14,000 rpm for 30 minutes at 4°C. The supernatant containing the solubilized protein can now be used “as is” or further purified. Further purification is recommended, as it will reduce the nonspecific binding and allow the direct quantification of how much CCR5 is attached to the sensor surface. To purify further, the retained soluble fraction is then mixed with 0.5 ml of 1D4-coated Sepharose beads (prepared by using the Affi-Gel Hz Immunoaffinity Kit). This suspension is rocked on a shaker overnight at 4°C. The following day, the beads are washed three times with 1 ml of a solution containing 100 mm (NH4)2SO4, 20 mm Tris-HCl, 10% glycerol, and 0.1% Cymal-5 or 1% CHAPSO, followed by one wash with 1 ml of a solution containing 100 mm (NH4)2SO4, 20 mm Tris-HCl, 10% glycerol, 100 mm MgCl2, and 0.1% Cymal-5 or 1% CHAPSO. Finally, elution of the solubilized receptor is achieved by using two 0.5-ml fractions of a solution containing 100 mm (NH4)2SO4, 20 mm Tris-HCl, 10% glycerol, and 0.1% Cymal-5 or 1% CHAPSO plus the synthesized TETSQVAPA peptide of 200 μm. The two eluted fractions of receptor are then pooled and dialyzed overnight into a solution of HBS-N and 0.5% Cymal-5 or 0.5% CHAPSO. CXCR4 is purified in a similar fashion.
Preparation of Lipid/Cholesterol/Detergent-mixed Micelle
To prepare lipid/detergent-mixed micelles, aliquots that represent 10 mg of DMPC dissolved in chloroform are placed into glass vials. A thin DMPC film is formed on the glass wall by rotating the tube while evaporating the chloroform. The lipids are then resolubilized by the addition of 3 mm CHAPSO or 2 mm Cymal-5 in 4.0 ml of HBS-N. These detergent concentrations were previously determined to be optimum for establishing a bilayer by testing capture levels on an underivatized L1 chip. A bilayer is generally thought to have formed upon deposition of between 4000 and 4600 RUs of lipid at the sensor surface (Nollert et al. 1995).
Immobilization of 1D4 on an L1 Chip
A number of different immobilization chemistries exist that are suitable for use with a surface plasmon resonance detector (O’Shannessy et al. 1992). The choice of immobilization strategy ultimately depends on the nature of the ligand to be immobilized. However, in general, oriented immobilization strategies tend to yield surfaces with increased activity over those in which the immobilization is random (Wilchek and Miron 2003). A strategy that can be used for the oriented immobilization of antibodies or other glycoproteins is aldehyde immobilization. This method again uses the formation of NHS esters from a fraction of the carboxyl groups of the CM-dextran matrix via reaction with NHS and EDC hydrochloride in H2O. However, this active ester is then converted to a hydrazine derivative by passing carbohydrazide across the surface. Any unreacted remaining active esters are converted into amides via reaction with ethanolamine, leaving only the hydrazine derivative available for reaction. This step eliminates the probability of coupling of the ligand by amine chemistry. The hydrazine derivative group can then participate in a reaction with native or introduced (e.g., oxidiation of the cis-diols of the carbohydrate moieties by using sodium periodate) aldehyde groups present on the ligand to be immobilized forming a hydrazone bond. Finally, the relatively unstable hydrazone bond is converted to a secondary amine group by exposure to sodium cyanoborohydride. In the case of an antibody, this mode of attachment results in a surface in which all of the antibodies are attached to the matrix by their Fc portions. Outlined below is a method to oxidize and couple an antibody via aldehyde chemistry to the carboxymethyldextran surface of a sensor chip.
Oxidation of the Carbohydrates on 1D4
Prepare a cold (on ice) solution of 1D4 in 100 mm sodium acetate buffer (pH 5.5) at a concentration of 1 mg ml–1.
To this solution, add sodium metaperiodate to a final concentration of 1 mm.
Incubate on ice for 20 minutes.
Stop the reaction by desalting the mixture on a NAP-5 column, followed by elution in 10 mm sodium acetate buffer (pH 4.0). Store the oxidized antibody at 4°C until needed.
Aldehyde Attachment of 1D4 to L1 Surface
Dock and prime the L1 chip with HBS-N as described in Protocol 1.1, for flow cell 1. Condition the chip surface with three short injections of octyl-β-glucoside to remove any contaminants that may hinder the coupling procedure.
Mix equal volumes (50 μl) of EDC and NHS stock solutions to a final concentration of 200 mm EDC/50 mm NHS.
Inject 35 μl of EDC/NHS solution to activate the sensor chip surface.
Inject 35 μl of 5 mm carbohydrazide, followed by 40 μl of ethanolamine, to block any sites that have not reacted with the carbohydrazide.
Inject 50 μl of the oxidized 1D4.
Lower the flow rate to 2 μl min–1 and inject 40 μl of 100 mm cyanoborohydride to stabilize the hydrazone bond formed during aldehyde coupling.
Repeat above steps on the separate flow cell 2. This will serve as a surface to affinity capture a similarly C9-tagged receptor such as CXCR4 from Cf2Th/synCXCR4 (see below). Additionally, oxidize the irrelevant mAb 2B6R and attach to flow cell 3, to create a second reference cell. This surface will allow quantification of how much tagged receptor is bound to the sensor chip.
Affinity Capture and Reconstitution of CCR5
Reconstitution of CCR5 is performed over a 1D4 surface at 25°C with HBS-N as the running buffer and at a flow rate of 10 μl min–1.
Inject 80 μl of solubilized/purified CCR5.
Inject 50 μl of 1 mm cholesterol in HBS-N.
Inject 80 μl of 3.4 mm DMPC/2 mm CHAPSO (or 3 mm Cymal-5).
Increase flow rate to 50 μl min–1.
Inject three 1-minute injections of sterile H2O to further promote bilayer formation.
Two reference cells are prepared: (1) one exactly as above except for the capture and lipid reconstitution of CXCR4 instead of CCR5 and (2) solubilized CCR5 followed by lipid passed across a surface with 2B6R.
Binding of Monoclonal Antibodies to Reconstituted CCR5
Binding assays are performed using reconstituted CCR5, at 25°C with PBS as the running buffer and at a flow rate of 50 μl min–1. A set concentration of monoclonal antibody (400 nm) or the chemokine RANTES (200 nm) is passed across the surfaces for a 1-minute association phase and a 2-minute dissociation phase. Regeneration is achieved by pulses of 35 mm NaOH/1.3 m NaCl (data shown in Fig. 15).
SPR Data Analysis
To obtain sensorgrams that represent the specific binding of the mAb-CCR5 interaction or the RANTES-CCR5 interaction, a double-reference subtraction is performed as outlined in Protocol 1.1, after which the sensorgrams can be analyzed using BIAevaluation software to obtain kinetic constants.
SECTION 3: INTEGRATION OF SPR BIOSENSORS WITH MASS SPECTROMETRY
The combination of SPR biosensors with mass spectrometry (MS) was first demonstrated in 1996 (Krone et al. 1996) and was subsequently described in several proof-of-principle articles (Krone et al. 1997; Nelson et al. 1997a). Recently, there has been increased interest in the SPR-MS approach (Fig. 16), and several groups have advanced the technology into new applications (Zhukov et al. 2002; Kikuchi et al. 2003; Lopez et al. 2003; Nedelkov and Nelson 2003a,b; Nedelkov et al. 2003). Both SPR biosensors and matrix-assisted laser desorption ionization–time of flight (MALDI-TOF) mass spectrometers have been commercially available since the early 1990s, and the combination of the two was the next logical step forward. The synergy of the two techniques offers unequivocal advantages: SPR is employed for quantification, whereas MS is utilized to delineate the structural features of the analyzed proteins. Proteins are initially affinity-captured from solution via ligands covalently attached on the SPR sensor surface. Quantification is achieved via SPR detection, which is nondestructive. Hence, the proteins retrieved on the SPR sensing surface can be further analyzed via MS, either directly from the sensor/chip surface (Nedelkov et al. 2000; Nedelkov and Nelson 2001a,b; Natsume et al. 2002) or separately, following an elution and microrecovery (Sonksen et al. 1998, 2001; Nelson et al. 1999; Gilligan et al. 2002). MS analysis offers a unique opportunity to delineate nonspecific binding (NSB) of other biomolecules to the surface-immobilized biomolecules (or to the underivatized sensor surface itself), binding of protein fragments, protein variants (existing due to posttranslational modifications and point mutations), and complexed (with other molecules/proteins). SPR sensing alone cannot detect any of the above events, due to its nondiscriminatory character (i.e., detection of all binding via mass change on the sensor surface).
Outline of Procedure
Any BIAcore (BIAcore AB) SPR biosensor can be utilized for protein affinity retrieval and SPR analysis (several other manufacturers of SPR biosensors are, most notably, Applied Biosystems and GWC Technologies, Inc.). In the current BIAcore instruments configuration, up to four sites (flow cells) can be used for simultaneous interrogation of binding events. Prior to insertion of the chip into the MS instrument, the sensor chip is removed from the plastic housing cassette and washed with several aliquots of ultrapure H2O, to remove any residual stabilizers that might interfere with the MS analysis. Ligands (e.g., antibodies) are generally immobilized on the CM-dextran surface of a CM5 Research-grade Sensor Chip (BIAcore AB, Cat. No. BR-1000-14) using an amine coupling kit (BIAcore AB, Cat. No. BR-1000-50). There are number of other surfaces (streptavidin, NTA, etc.) that can also be utilized to immobilize the ligands. However, noncovalent affinity-ligand attachment is not preferred, because during the MS analysis from the chip, signals from both the ligand and the captured analyte will appear in the mass spectra, as covalently attached ligands stay bound to the surface during the MALDI process and are not registered by the MS detector.
When using antibodies (or other proteins) as ligands, the original stock solutions are diluted with low-to-medium pH buffer (e.g., 10 mm acetate buffer at pH 5.0), to a final ligand concentration of 0.01–0.1 mg ml–1. Contrary to typical kinetic SPR analysis, high ligand (~100–200 fmole mm–2 for antibodies) densities should be achieved on the sensor surface so that ample amounts of analyte are captured for subsequent MALDI-TOF MS analysis. Following ligand immobilization, solutions containing the analyte (e.g., protein) of interest are injected over the flow cells. Lower flow rates (5–10 μl min–1, promoting mass transfer effects) are utilized to increase the binding of the analyte to the immobilized ligands. At high flow rates (60–100 μl min–1) low-concentration analytes will not be captured in amounts permissive of downstream MS analysis.
Following the sample analysis, analyte retrieval, and SPR signal reading, the chip is promptly removed from the biosensor to avoid losses of the captured proteins (especially when the interaction system under study exhibits a fast dissociation phase). For the same reasons, lengthy postcapture washes of the chip should be avoided, although several quick rinses with 200 μl aliquots of ultrapure H2O are highly recommended to remove any residual buffer components that will interfere with MS analysis.
Once removed from the biosensor, the chip is prepared for MS. We have used, in the past, and with great success, a chip cutter with a circular heated cutter-head (Intrinsic Bioprobes Inc.) for excising a chip/plastic mount of a defined circular shape that fits into an appropriately configured MALDI mass spectrometer target (Nedelkov and Nelson 2000). However, most commercially available mass spectrometers can accommodate the large footprint of the entire chip-housing, as long as the MALDI target plates are machined/milled to accommodate the entire chip.
The MALDI matrix is applied to the chip via an aerosol-spraying device (Intrinsic Bioprobes Inc.). The device consists of an aspirating/sheath gas needle (~30 μm orifice), backed by approximately 30 psi of compressed air (Nedelkov and Nelson 2000). The air/matrix solution ratio can be adjusted to produce a fine mist of matrix solution that is aimed at the entire surface of the cutout chip. The matrix mist should moisten, but not completely wet, the chip surface (i.e., the tiny matrix droplets should stay as individual drops on the surface and not be connected into one large liquid drop). The matrix droplets desorb the proteins from their respective capturing affinity ligand, and, upon rapid drying, the matrix/protein mixture is redeposited on the same area where the proteins were originally captured in the SPR analysis.
In most MS experiments, higher-quality mass spectra are obtained when α-cyano-4-hydroxycinnamic acid (ACCA) is used as a MALDI matrix for proteins smaller than approximately 25 kD. In contrast, sinapic acid (SA) yields better results for higher-molecular-mass (>25 kD) proteins. For the MS analysis from the SPR chips, however, ACCA is superior, in that it is a better energy-absorbing matrix and, consequently, requires less laser power. Lower laser power means that more spectra can be obtained from a single spot, and fast burning through the matrix/sample layer is avoided (i.e., the analyte is captured in a very thin layer on the surface of the chip, and aggressive interrogation with the laser at high frequency can rapidly deplete the sample spot). Re-application of more matrix (following initial application and MS analysis) does not yield better signals and generally results in decreased signal-to-noise ratio.
The SPR-MS approach is becoming very valuable in studying protein interactions from biological solutions. The MS dimension of the analysis examines the structural state of the interacting molecules, readily revealing the existence of posttranslational modifications. SPR-MS analysis can be performed on any protein for which there is an interacting partner that can be immobilized on the chip surface. SPR-MS yields ample data for both small and large proteins, although signals from proteins above about 100 kD in mass are typically harder to delineate in the mass spectra (due to factors such as resolution and ionization efficiency). With respect to the concentration range, proteins with a concentration of 1 ng ml–1 in complex biological fluids have been detected via SPR-MS, translating into retrieval and detection of hundreds of attomoles (1018 moles) of protein on the chip surface (Nedelkov and Nelson 2000b, 2003c). It is important to note that the limit of detection is highly dependent on the affinity of the interaction (i.e., the amount of protein captured on the chip surface), as that is the material registered by the SPR sensing, and thus the MALDI-TOF mass spectrometry analysis. In addition to antibodies, other proteins can also be used as ligands for protein retrieval and detection (Nedelkov and Nelson 2003a). Small molecules can also be immobilized and used in preliminary target binding screens.
In sum, the combination of SPR with MS creates a unique platform for complete protein analysis. The platform can readily be expanded to accommodate the new SPR array sensors that are coming to the market, resulting in rapid and high-throughput screening of biological samples for protein functionalities. Such SPR-MS arrays would enable multiplexed quantitative and qualitative functional analyses of hundreds of proteins from small amounts of samples. An example of the results is given in Figure 17.
Protocol 3.1: SPR-MS Analysis
This protocol outlines the procedure of concerted SPR-MS analysis. The example given is the analysis of C-reactive protein (Zimmerman et al. 2003) from human plasma via surface-immobilized anti-CRP polyclonal antibody.
MATERIALS
Caution: See Appendix for appropriate handling of materials marked with <!>.
Buffers and Reagents
Refer to Protocol 1.1 for standard reagents
10 mm HEPES (pH 7.4), 0.15 m NaCl, 0.005% (v/v) surfactant P20, 3 mm EDTA (HBS-EP; BIAcore AB)
Acetonitrile (Sigma) <!>
Trifluoroacetic acid (TFA; Sigma) <!>
α-Cyano-4-hydroxycinnamic acid (ACCA; Sigma) <!>
Carbon paint (SPI Supplies)
Proteins
Anti-CRP polyclonal antibody (DAKO Cytomation, Cat. No. A0073)
CRP standard (Calbiochem, Cat. No. 236603)
Special Equipment
Refer to Protocol 1.1
BiacoreX Optical Biosensor (BIAcore AB)
Circular heated head-cutter saw (Intrinsic Bioprobes Inc.)
MALDI-TOF mass spectrometer with the target configured to accommodate a specific piece of the chip excised using the cutter saw
METHODS
Immobilization of Anti-CRP Antibody to Sensor Chip
Immobilization of the anti-CRP antibody to flow cells 1 and 2 of a CM5 chip is accomplished using the standard amine-coupling procedure outlined in Protocol 1.1. Anti-CRP antibody (16 mg-ml–1) is diluted 100-fold in coupling buffer (10 mm sodium acetate at pH 5.0) and injected until the surface density is about 16,500 RUs.
Preparation of Human Plasma Sample
Blood (45 μl) is drawn under sterile conditions from a lancet-punctured finger with heparinized microcolumn (Drummond Scientific Co.), mixed with 200 μl of HBS-EP buffer, and centrifuged for 30 seconds (at 7000 RPM, 2500g) to pellet the red blood cells. The supernatant (plasma) is then collected and used as is for the injection.
Affinity Capture of CRP
The two derivatized flow cells are equilibrated in buffer. The flow is switched to flow cell 2 (FC2), and a single 500-μl aliquot of 10–3 mg ml–1 of CRP standard is injected. This allows the determination of functionality of the antibody surface and also serves as a control for CRP with minimal plasma component contamination. The flow is then switched to flow cell 1 (FC1), and a 50-μl aliquot of tenfold diluted fresh human plasma is then injected.
Preparation of Sensor Chip, Matrix Application, and MS Analysis
Following injection and affinity capture of CRP from the purified standard and from diluted human plasma, the buffer flow is stopped, and the chip is removed from the sensor. The chip is then washed three times with 200 μl of ultrapure, sterile H2O. The chip is allowed to dry, and transparent tape is placed on the back of the chip to prevent accidental breakage during cutting. The chip is then cut using the heated head cutter, and a saturated aqueous solution of MALDI matrix (ACCA, in 33% [v/v] acetonitrile, 0.2% [v/v] TFA) is then applied using the aerosol delivery system. Upon drying of the matrix, the chip is then placed onto a mass spectrometer target holder, and carbon paint is applied on the chip-target interface to provide a conductive contact.
MS is performed using a homemade MALDI-TOF mass spectrometer, details of which can be found in Nelson et al. (1997b). Each site/flow cell on the sensor chip is targeted individually with the nitrogen laser, allowing site-specific BIA/MS analysis. The mass spectra are then acquired in the positive ion mode.
APPENDIX: CAUTIONS
Please note that the Cautions Appendix in this manual is not exhaustive. Readers should always consult individual manufacturers and other resources for current and specific product information. Chemicals and other materials discussed in text sections are not identified by the icon (!) used to indicate hazardous materials in the protocols. However, they may be hazardous to the user without special handling. Please consult your local safety office or the manufacturer’s safety guidelines for further information.
The following general cautions should always be observed.
Become completely familiar with the properties of substances used before beginning the procedure.
The absence of a warning does not necessarily mean that the material is safe, because information may not always be complete or available.
If exposed to toxic substances, contact your local safety office immediately for instructions.
Use proper disposal procedures for all chemical, biological, and radioactive waste.
For specific guidelines on appropriate gloves, consult your local safety office.
Handle concentrated acids and bases with great care. Wear goggles and appropriate gloves. A face shield should be worn when handling large quantities.
Do not mix strong acids with organic solvents as they may react. Sulfuric acid and nitric acid especially may react highly exothermically and cause fires and explosions.
Do not mix strong bases with halogenated solvent as they may form reactive carbenes which can lead to explosions.
Handle and store pressurized gas containers with caution as they may contain flammable, toxic, or corrosive gases; asphyxiants; or oxidizers. For proper procedures, consult the Material Safety Data Sheet that must be provided by your vendor.
Never pipette solutions using mouth suction. This method is not sterile and can be dangerous. Always use a pipette aid or bulb.
Keep halogenated and nonhalogenated solvents separately (e.g., mixing chloroform and acetone can cause unexpected reactions in the presence of bases). Halogenated solvents are organic solvents such as chloroform, dichloromethane, trichlorotrifluoroethane, and dichloroethane. Some nonhalogenated solvents are pentane, heptane, ethanol, methanol, benzene, toluene, N,N-dimethylformamide (DMF), dimethyl sulfoxide (DMSO), and acetonitrile.
Laser radiation, visible or invisible, can cause severe damage to the eyes and skin. Take proper precautions to prevent exposure to direct and reflected beams. Always follow manufacturer’s safety guidelines and consult your local safety office. See caution below for more detailed information.
Flash lamps, due to their light intensity, can be harmful to the eyes. They also may explode on occasion. Wear appropriate eye protection and follow the manufacturer’s guidelines.
Photographic fixatives and developers also contain chemicals that can be harmful. Handle them with care and follow manufacturer’s directions.
Power supplies and electrophoresis equipment pose serious fire hazard and electrical shock hazards if not used properly.
Microwave ovens and autoclaves in the lab require certain precautions. Accidents have occurred involving their use (e.g., to melt agar or bacto-agar stored in bottles or to sterilize). If the screw top is not completely removed and there is not enough space for the steam to vent, the bottles can explode and cause severe injury when the containers are removed from the microwave or autoclave. Always completely remove bottle caps before microwaving or autoclaving. An alternative method for routine agarose gels that do not require sterile agar is to weigh out the agar and place the solution in a flask.
Ultrasonicators use high frequency sound waves (16 - 100 kHz) for cell disruption and other purposes. This "ultrasound," conducted through air, does not pose a direct hazard to humans, but the associated high volumes of audible sound can cause a variety of effects, including headache, nausea, and tinnitus. Direct contact of the body with high-intensity ultrasound (not medical imaging equipment) should be avoided. Use appropriate ear protection and display signs on the door(s) of laboratories where the units are used.
Use extreme caution when handling cutting devices such as microtome blades, scalpels, razor blades, or needles. Microtome blades are extremely sharp! Use care when sectioning. If unfamiliar with their use, have someone demonstrate proper procedures. For proper disposal, use the “sharps” disposal container in your lab. Discard used needles unshielded, with the syringe still attached. This prevents injuries (and possible infections; see Biological Safety) while manipulating used needles because many accidents occur while trying to replace the needle shield. Injuries may also be caused by broken Pasteur pipettes, coverslips, or slides.
Acetic acid (glacial) is highly corrosive and must be handled with great care. Liquid and mist cause severe burns to all body tissues. It may be harmful by inhalation, ingestion, or skin absorption. Wear appropriate gloves and goggles and use in a chemical fume hood. Keep away from heat, sparks, and open flame.
Acetonitrile (Methyl cyanide) is very volatile and extremely flammable. It is an irritant and a chemical asphyxiant that can exert its effects by inhalation, ingestion, or skin absorption. Treat cases of severe exposure as cyanide poisoning. Wear appropriate gloves and safety glasses and use only in a chemical fume hood. Keep away from heat, sparks, and open flame.
Citric acid is an irritant and may be harmful by inhalation, ingestion, or skin absorption. It poses a risk of serious damage to the eyes. Wear appropriate gloves and safety goggles. Do not breathe the dust.
α-Cyano-4-hydroxycinnamic acid may cause cardiac disturbances. Chronic effects may be delayed. It may be harmful by inhalation, ingestion, or skin absorption. Wear appropriate gloves and safety glasses.
Cysteine is an irritant to the eyes, skin, and respiratory tract. It may be harmful by inhalation, ingestion, or skin absorption. Wear appropriate gloves and safety glasses. Do not breathe the dust.
Cysteine hydrochloride is an irritant to the eyes, skin, and respiratory tract. It may be harmful by inhalation, ingestion, or skin absorption. Wear appropriate gloves and safety glasses. Do not breathe the dust.
DCM, see Dichloromethane
Dichloromethane (DCM), CH2Cl2, (also known as Methylene chloride) is toxic if inhaled, ingested, or absorbed through the skin. It is also an irritant and is suspected to be a carcinogen. Wear appropriate gloves and safety glasses and use in a chemical fume hood. Do not breathe the vapors.
DIEA, see Diisopropylethylamine
Diisopropylethylamine (DIEA) is extremely destructive to the mucous membranes, upper respiratory tract, skin, and eyes. It may be harmful by ingestion or skin absorption. Inhalation may be fatal. Wear appropriate gloves and safety glasses and always use in a chemical fume hood. Keep away from heat sparks, and open flame.
EDC, see N-ethyl-N′-(dimethylaminopropyl)-carbodiimide
Ethanedithiol is toxic and harmful by inhalation, ingestion, or skin absorption. Handle with care and avoid any contact with skin. Wear appropriate gloves and goggles and use only in a chemical fume hood.
Ethanolamine, HOCH2CH2NH2, is toxic and harmful by inhalation, ingestion, or skin absorption. Handle with care and avoid any contact with the skin. Wear appropriate gloves and goggles and use in a chemical fume hood. Ethanolamine is highly corrosive and reacts violently with acids.
Glycine may be harmful by inhalation, ingestion, or skin absorption. Wear gloves and safety glasses. Avoid breathing the dust.
2-[1H-benzotriazol-1-yl]-1,1,3,3-tetramethyluronium hexafluorophosphate (HBTU) is irritating to the eyes, skin, mucous membranes, and upper respiratory tract. It may be harmful by inhalation, ingestion, or skin absorption. Wear appropriate gloves and safety glasses and do not breathe the dust.
HBTU, see 2-[1H-benzotriazol-1-yl]-1,1,3,3-tetramethyluronium hexafluorophosphate
HCl, see Hydrochloric acid
H3COH, see Methanol
HOBT, see 1-Hydroxybenzotriazole
HOCH2CH2NH2, see Ethanolamine
Hydrochloric acid, HCl, is volatile and may be fatal if inhaled, ingested, or absorbed through the skin. It is extremely destructive to mucous membranes, upper respiratory tract, eyes, and skin. Wear appropriate gloves and safety glasses and use with great care in a chemical fume hood. Wear goggles when handling large quantities.
1-Hydroxybenzotriazole (HOBT) and N-Hydroxybenzotriazole are highly flammable and irritating to the eyes, skin, and mucous membranes. They may be toxic by inhalation, ingestion, or skin absorption. Wear appropriate gloves and safety glasses and use in a chemical fume hood. Keep away from heat, sparks, and open flame.
Hygromycin B is highly toxic and may be fatal if inhaled, ingested, or absorbed through the skin. Wear appropriate gloves and safety goggles and use only in a chemical fume hood. Do not breathe the dust.
MeOH or H3COH, see Methanol
Methanol, MeOH or H3COH, is poisonous and can cause blindness. It may be harmful by inhalation, ingestion, or skin absorption. Adequate ventilation is necessary to limit exposure to vapors. Avoid inhaling these vapors. Wear appropriate gloves and goggles and use only in a chemical fume hood.
Methyl cyanide, see Acetonitrile.
Methylene chloride, see Dichloromethane
NaOH, see Sodium hydroxide
NaOAc, see Sodium acetate
N-Ethyl-N′-(dimethylaminopropyl)-carbodiimide (EDC) is irritating to the mucous membranes and upper respiratory tract. It may be harmful by inhalation, ingestion, or skin absorption. Wear appropriate gloves and safety glasses. Handle with care.
N-Hydroxysuccinimide is an irritant and may be harmful by inhalation, ingestion, or skin absorption. Wear appropriate gloves and safety glasses.
N-Methyl-2-pyrrolidinone, 2-Methyl-2-pyrrolidinone, and 1-Methyl-2-pyrrolidinone) are combustible and may be harmful by inhalation, ingestion, or skin absorption. Wear appropriate gloves and safety glasses and use in a chemical fume hood. Do not breathe the vapors. Keep away from heat, sparks, and open flame.
Piperidine is highly toxic and is corrosive to the eyes, skin, respiratory tract, and gastrointestinal tract. It reacts violently with acids and oxidizing agents and may be harmful by inhalation, ingestion, or skin absorption. Do not breathe the vapors. Keep away from heat, sparks, and open flame. Wear appropriate gloves and safety glasses and use in a chemical fume hood.
Pluronic acid may be harmful by inhalation, ingestion, or skin absorption. Wear appropriate gloves and safety glasses.
Sodium acetate (NaOAc), see Acetic acid
Sodium citrate, see Citric acid
Sodium hydroxide, NaOH, and solutions containing NaOH are highly toxic and caustic and should be handled with great care. Wear appropriate gloves and a face mask. All other concentrated bases should be handled in a similar manner.
Sodium phosphate, NaH2PO4/Na2HPO4/Na3PO4, is an irritant to the eyes and skin. It may be harmful by inhalation, ingestion, or skin absorption. Wear appropriate gloves and safety goggles. Do not breathe the dust.
Streptomycin is toxic and a suspected carcinogen and mutagen. It may cause allergic reactions. It may be harmful by inhalation, ingestion, or skin absorption. Wear appropriate gloves and safety glasses.
TFA, see Trifluoroacetic acid
Thioanisole is an irritant and may be harmful by inhalation, ingestion, or skin absorption. Wear appropriate gloves, face mask, and safety glasses and use only in a chemical fume hood. Do not breathe the vapors or fumes, which are toxic.
Trifluoroacetic acid (TFA) (concentrated) may be harmful by inhalation, ingestion, or skin absorption. Concentrated acids must be handled with great care. Decomposition causes toxic fumes. Wear appropriate gloves and a face mask and use in a chemical fume hood.
Xylazine may be harmful by inhalation, ingestion, or skin absorption. Wear appropriate gloves and safety glasses.
REFERENCES
Anderson N.L., Matheson A.D., and Steiner S. 2000. Proteomics: Applications in basic and applied biology. Curr. Opin. Biotechnol. 11: 408–412.
Babcock G.J., Mirzabekov T., Wojtowicz W., and Sodroski J. 2001. Ligand binding characteristics of CXCR4 incorporated into paramagnetic proteoliposomes. J. Biol. Chem. 276: 38433–38440.
Balzarini J., Pannecouque C., De Clercq E., Pavlov A.Y., Printsevskaya S.S., Miroshnikova O.V., Reznikova M.I., and Preobrazhenskaya M.N. 2003. Antiretroviral activity of semisynthetic derivatives of glycopeptide antibiotics. J. Med. Chem. 46: 2755–2764.
Banner D.W., Kokkinidis M., and Tsernoglou D. 1987. Structure of the ColE1 rop protein at 1.7 Å resolution. J. Mol. Biol. 196: 657–675.
Betz S., Fairman R., Fairman R., O’Neil K., Lear J., and Degrado W. 1995. Design of two-stranded and three-stranded coiled-coil peptides. Philos. Trans. R. Soc. Lond. B Biol. Sci. 348: 81–88.
Brigham-Burke M., Edwards J.R., and O’Shannessy D.J. 1992. Detection of receptor-ligand interactions using surface plasmon resonance: Model studies employing the HIV-1 gp120/CD4 interaction. Anal. Biochem. 205: 125–131.
Biorn A.C., Cocklin S., Madani N., Si Z., Ivanovic T., Samanen J., Van Ryk D.I., Pantophlet R., Burton D.R., Freire E., Sodroski J., and Chaiken I. 2004. Mode of action for linear peptide inhibitors of HIV-1 gp 120 interactions. Biochemistry 43: 1928–2131.
Burkhard P., Ivaninskii S., and Lustig A. 2002. Improving coiled-coil stability by optimizing ionic interactions. J. Mol. Biol. 318: 901–910.
Canziani G., Zhang W., Cines D., Rux A., Willis S., Cohen G., Eisenberg R., and Chaiken I.M. 1999. Exploring biomolecular recognition using optical biosensors. Methods 19: 253–269.
Chaiken I.M. 2001. Revealing and utilizing receptor recognition mechanisms in a high throughput world. J. Cell. Biochem. Suppl. 37: 126–135.
Chaiken I., Wu S.J., Plugariu C., Zhang W., Li C., and Dowd C. 2001. Convergence of recombinant mutagenesis and kinetics interaction analysis for revealing receptor recognition mechanisms and designing receptor ligands. In Drug-receptor thermodynamics: Introduction and applications (ed. R.B. Raffa), pp. 743–781. Wiley, Chichester, United Kingdom.
Clapham P.R. and McKnight A. 2002. Cell surface receptors, virus entry and tropism of primate lentiviruses. J. Gen. Virol. 83: 1809–1829.
Cohen C. and Parry D.A. 1990. Alpha-helical coiled coils and bundles: How to design an alpha-helical protein. Proteins 7: 1–15.
Crick F.H.C. 1953. The packing of alpha-helices: Simple coiled-coils. Acta Crystallogr. 6: 689.
Culp J.S., Johansen H., Hellmig B., Beck J., Matthews T.J., Delers A., and Rosenberg M. 1991. Regulated expression allows high level production and secretion of HIV-1 gp120 envelope glycoprotein in Drosophila Schneider cells. Biotechnology 9: 173–177.
Cusack S., Berthet-Colominas C., Hartlein M., Nassar N., and Leberman R. 1990. A second class of synthetase structure revealed by X-ray analysis of Escherichia coli seryl tRNA synthetase at 2.5 Å. Nature 347: 249–255.
De Crescenzo G., Pham P.L., Durocher Y., and O’Connor-McCourt M.D. 2003. Transforming growth factor-beta (TGF-β) binding to the extracellular domain of the type II TGF-β receptor: Receptor capture on a biosensor surface using a new coiled-coil capture system demonstrates that avidity contributes significantly to high affinity binding. J. Mol. Biol. 328: 1173–1183.
Dongre A.R., Opiteck G., Cosand W.L., and Hefta S.A. 2001. Proteomics in the post genome age. Biopolymers 60: 206–211.
Dowd C.S., Leavitt S., Babcock G., Godillot A.P., Van Ryk D., Canziani G.A., Sodroski J., Freire E., and Chaiken I.M. 2002. β-turn Phe in HIV-1 Env binding site of CD4 and CD4 mimetic miniprotein enhances Env binding affinity but is not required for activation of co-receptor/17b site. Biochemistry 41: 7038–7046.
Doyle A., Griffiths J.B., and Newell D.G., eds. 1998. Cell and tissue culture: Laboratory procedures. Wiley, United Kingdom.
Ferrer M. and Harrison S.C. 1999. Peptide ligands to human immunodeficiency virus type 1 gp120 identified from phage display libraries. J. Virol. 73: 5795–5802.
Foster P.S., Hogan S.P., Yang M., Mattes J., Young I.G., Matthaei K.I., Kumar R.K., Mahalingam S., and Webb D.C. 2002. Interleukin-5 and eosinophils as therapeutic targets for asthma. Trends Mol. Med. 8: 162–167.
Fredriksson R., Lagerstrom M.C., Lundin L.G., and Schioth H.B. 2003. The G-protein-coupled receptors in the human genome form five main families. Phylogenetic analysis, paralogon groups, and fingerprints. Mol. Pharmacol. 63: 1256–1272.
Gilligan J.J., Schuck P., and Yergey A.L. 2002. Mass spectrometry after capture and small-volume elution of analyte from a surface plasmon resonance biosensor. Anal. Chem. 74: 2041–2047.
Giot L., Bader J.S., Brouwer C., Chaudhuri A., Kuang B., Li Y., Hao Y.L., Ooi C.E., Godwin B., Vitols E., et al. 2003. A protein map of Drosophila melanogaster. Science 302: 1727–1736.
Hicks M.R., Holberton D.V., Kowalczyk C., and Woolfson D.N. 1997. Coiled-coil assembly by peptides with non-heptad sequence motifs. Fold. Des. 2: 149–158.
Hodges R.S., Zhou N.E., Kay C.M., and Semchuk P.D. 1990. Synthetic model proteins: Contribution of hydrophobic residues and disulfide bonds to protein stability. Pept. Res. 3: 123–137.
Hodges R.S., Heaton R.J., Parker J.M., Molday L., and Molday R.S. 1988. Antigen–antibody interaction. Synthetic peptides define linear antigenic determinants recognized by monoclonal antibodies directed to the cytoplasmic carboxyl terminus of rhodopsin. J. Biol. Chem. 263: 11768–11775.
Hong Q., Gutierrez-Aguirre I., Barlic A., Malovrh P., Kristan K., Podlesek Z., Macek P., Turk D., Gonzalez-Manas J.M., Lakey J.H., and Anderluh G. 2002. Two-step membrane binding by Equinatoxin II, a pore-forming toxin from the sea anemone, involves an exposed aromatic cluster and a flexible helix. J. Biol. Chem. 277: 41916–41924.
Howard K. 2000. The bioinformatics gold rush. Sci. Am. 283: 58–63.
Ishino T., Pasut G., Scibek J., and Chaiken I. 2004. Kinetic interaction analysis of human interleukin 5 receptor α mutants reveals a unique binding topology and charge distribution for cytokine recognition. J. Biol. Chem. 279: 9547–9556.
Johanson K., Appelbaum E., Doyle M., Hensley P., Zhao B., Abdel-Meguid S.S., Young P., Cook R., Carr S., Matico R., Cusimano D., Dul E., Angelichio M., Brooks I., Winborne E., McDonnell P., Morton T., Bennett D., Sokoloski T., McNulty D., Rosenberg M., and Chaiken I. 1995. Binding interactions of human interleukin 5 with its receptor α subunit. J. Biol. Chem. 270: 9459–9471.
Johnsson B., Lofas S., and Lindquist G. 1991. Immobilization of proteins to a carboxymethyldextran-modified gold surface for biospecific interaction analysis in surface plasmon resonance sensors. Anal. Biochem. 198: 268–277.
Jones P.L., Korte T., and Blumenthal R. 1998. Conformational changes in cell surface HIV-1 envelope glycoproteins are triggered by cooperation between cell surface CD4 and co-receptors. J. Biol. Chem. 273: 404–409.
Karlen S., De Boer M.L., Lipscombe R.J., Lutz W., Mordinov V.A., and Sanderson C.J. 1998. Biological and molecular characteristics of interleukin-5 and its receptor. Int. Rev. Immunol. 16: 227–247.
Karlsson O.P. and Lofas S. 2002. Flow-mediated on-surface reconstitution of G-protein coupled receptors for applications in surface plasmon resonance biosensors. Anal. Biochem. 300: 132–138.
Kikuchi J., Furukawa Y., and Hayashi N. 2003. Identification of novel p53-binding proteins by biomolecular interaction analysis combined with tandem mass spectrometry. Mol. Biotechnol. 23: 203–212.
Koyanagi Y., Miles S., Mitsuyasu R.T., Merrill J.E., Vinters H.V., and Chen I.S.Y. 1987. Dual infection of the central nervous system by AIDS viruses with distinct cellular tropisms. Science 236: 819–822.
Krone J.R., Nelson R.W., Dogruel D., Williams P., and Granzow R. 1996. Interfacing mass spectrometric immunoassays with BIA. BIAJournal 3: 16–17.
_______. 1997. BIA/MS: Interfacing biomolecular interaction analysis with mass spectrometry. Anal. Biochem. 244: 124–132.
Kwong P.D., Doyle M.L., Casper D.J., Cicala C., Leavitt S.A., Majeed S., Steenbeke T.D., Venturi M., Chaiken I., Fung M., Katinger H., Parren P.W., Robinson J., Van Ryk D., Wang L., Burton D.R., Freire E., Wyatt R., Sodroski J., Hendrickson W.A., and Arthos J. 2002. HIV-1 evades antibody-mediated neutralization through conformational masking of receptor-binding sites. Nature 420: 678–682.
Li Y., Kappes J.C., Conway J.A., Price R.W., Shaw G.M., and Hahn B.H. 1991. Molecular characterization of human immunodeficiency virus type 1 cloned directly from uncultured human brain tissue: Identification of replication-competent and -defective viral genomes. J. Virol. 65: 3973–3985.
Lopez F., Pichereaux C., Burlet-Schiltz O., Pradayrol L., Monsarrat B., and Esteve J.P. 2003. Improved sensitivity of biomolecular interaction analysis mass spectrometry for the identification of interacting molecules. Proteomics 3: 402–412.
Lovejoy B., Choe S., Cascio D., McRorie D.K., DeGrado W.F., and Eisenberg D. 1993. Crystal structure of a synthetic triple-stranded alpha-helical bundle. Science 259: 1288–1293.
Martinez-Moczygemba M. and Huston D.P. 2003. Biology of common β receptor-signaling cytokines: IL-3, IL-5, and GM-CSF. J. Allergy Clin. Immunol. 112: 653–665.
Mirzabekov T., Bannert N., Farzan M., Hofmann W., Kolchinsky P., Wu L., Wyatt R., and Sodroski J. 1999. Enhanced expression, native purification, and characterization of CCR5, a principal HIV-1 coreceptor. J. Biol. Chem. 274: 28745–28750.
Morton T., Li J., Cook R., and Chaiken I. 1995. Mutagenesis in the C-terminal region of human interleukin 5 reveals a central patch for receptor alpha chain recognition. Proc. Natl. Acad. Sci. 92: 10879–10883.
Murata Y., Takaki S., Migita M., Kikuchi Y., Tominaga A., and Takatsu K. 1992. Molecular cloning and expression of the human interleukin 5 receptor. J. Exp. Med. 175: 341–351.
Myszka D.G. 1999. Improving biosensor analysis. J. Mol. Recognit. 12: 279–284.
Myszka D.G., Sweet R.W., Hensley P., Brigham-Burke M., Kwong P.D., Hendrickson W.A., Wyatt R., Sodroski J., and Doyle M.L. 2000. Energetics of the HIV gp120-CD4 binding reaction. Proc. Natl. Acad. Sci. 97: 9026–9031.
Natsume T., Taoka M., Manki H., Kume S., Isobe T., and Mikoshiba K. 2002. Rapid analysis of protein interactions: On-chip micropurification of recombinant protein expressed in Esherichia coli. Proteomics 2: 1247–1253.
Nedelkov D. and Nelson R.W. 2000a. Practical considerations in BIA/MS: Optimizing the biosensor-mass spectrometry interface. J. Mol. Recognit. 13: 140–145.
_______. 2000b. Exploring the limit of detection in biomolecular interaction analysis mass spectrometry (BIA/MS): Detection of attomole amounts of native proteins present in complex biological mixtures. Anal. Chim. Acta 423: 1–7.
_______. 2001a. Analysis of human urine protein biomarkers via biomolecular interaction analysis mass spectrometry. Am. J. Kidney Dis. 38: 481–487.
_______. 2001b. Delineation of in vivo assembled multiprotein complexes via biomolecular interaction analysis mass spectrometry. Proteomics 1: 1441–1446.
_______. 2003a. Delineating protein-protein interactions via biomolecular interaction analysis-mass spectrometry. J. Mol. Recognit. 16: 9–14.
_______. 2003b. Design and use of multi-affinity surfaces in biomolecular interaction analysis-mass spectrometry (BIA/MS): A step toward the design of SPR/MS arrays. J. Mol. Recognit. 16: 15–19.
_______. 2003c. Detection of Staphylococcal enterotoxin B via biomolecular interaction analysis mass spectrometry. Appl. Environ. Microbiol. 69: 5212–5215.
Nedelkov D., Rasooly A., and Nelson R.W. 2000. Multitoxin biosensor-mass spectrometry analysis: A new approach for rapid, real-time, sensitive analysis of staphylococcal toxins in food. Int. J. Food Microbiol. 60: 1–13.
Nedelkov D., Nelson R.W., Kiernan U.A., Niederkofler E.E., and Tubbs K.A. 2003. Detection of bound and free IGF-1 and IGF-2 in human plasma via biomolecular interaction analysis mass spectrometry. FEBS Lett. 536: 130–134.
Nelson R.W, Krone J.R., and Jansson O. 1997a. Surface plasmon resonance biomolecular interaction analysis mass spectrometry. 1. Chip-based analysis. Anal. Chem. 69: 4363–4368.
Nelson R.W., Jarvik J.W., Taillon B.E., and Tubbs K.A. 1999. BIA/MS of epitope-tagged peptides directly from E. coli lysate: Multiplex detection and protein identification at low-femtomole to subfemtomole levels. Anal. Chem. 71: 2858–2865.
Nelson R.W., Krone J.R., Dogruel D., Tubbs K.A., Granzow R., and Jansson O. 1997b. Techniques in protein chemistry VIII, pp. 493–504. Academic Press, San Diego.
Nollert P., Kiefer H., and Jahnig F. 1995. Lipid vesicle adsorption versus formation of planar bilayers on solid surfaces. Biophys. J. 69: 1447–1455.
Oas T.G., McIntosh L.P., O’Shea E.K., Dahlquist F.W., and Kim P.S. 1990. Secondary structure of a leucine zipper determined by nuclear magnetic resonance spectroscopy. Biochemistry 29: 2891–2894.
O’Shannessy D.J., Brigham-Burke M., and Peck K. 1992. Immobilization chemistries suitable for use in the BIAcore surface plasmon resonance detector. Anal. Biochem. 205: 132–136.
O’Shea E.K., Klemm J.D., Kim P.S., and Alber T. 1991. X-ray structure of the GCN4 leucine zipper, a two-stranded, parallel coiled coil. Science 254: 539–544.
Palczewski K., Kumasaka T., Hori T., Behnke C.A., Motoshima H., Fox B.A., Le-Trong I., Teller D.C., Okada T., Stenkamp R.E., Yamamoto M., and Miyano M. 2000. Crystal structure of rhodopsin: A G protein-coupled receptor. Science 289: 739–745.
Sattentau Q.J. and Moore J.P. 1991. Conformational changes induced in the human immunodeficiency virus envelope glycoprotein by soluble CD4 binding. J. Exp. Med. 174: 407–415.
Sattentau Q.J., Moore J.P., Vignaux F., Traincard F., and Poignard P. 1993. Conformational changes induced in the envelope glycoproteins of the human and simian immunodeficiency viruses by soluble receptor binding. J. Virol. 67: 7383–7393.
Scibek J.J., Evergren E., Zahn S., Canziani G.A., Van Ryk D., and Chaiken I.M. 2002. Biosensor analysis of dynamics of interleukin 5 receptor subunit βc interaction with IL5:IL5Rα complexes. Anal. Biochem. 307: 258–265.
Sergei M., Zurawski J., Cocklin S., and Chaiken I. 2004. Proteins, recognition networks and developing interfaces for macromolecular biosensing. J. Mol. Recognit. 17: 198–208.
Si Z., Madani N., Cox J.M., Chruma J.J., Klein J.C., Schon A., Phan N., Wang L., Biorn A.C., Cocklin S., Chaiken I., Freire E., Smith A.B., III, and Sodroski J.G. 2004. Small-molecule inhibitors of HIV-1 entry block receptor-induced conformational changes in the viral envelope glycoproteins. Proc. Natl. Acad. Sci. 101: 5036–5041.
Sonksen C.P., Nordhoff E., Jansson O., Malmqvist M., and Roepstorff P. 1998. Combining MALDI mass spectrometry and biomolecular interaction analysis using a biomolecular interaction analysis instrument. Anal. Chem. 70: 2731–2736.
Sonksen C.P., Roepstorff P., Markgren P.O., Danielson U.H., Hamalainen M.D., and Jansson O. 2001. Capture and analysis of low molecular weight ligands by surface plasmon resonance combined with mass spectrometry. Eur. J. Mass Spectrom. 7: 385–391.
Stenlund P., Babcock G.J., Sodroski J., and Myszka D.G. 2003. Capture and reconstitution of G protein-coupled receptors on a biosensor surface. Anal. Biochem. 316: 243–250.
Sullivan N., Sun Y., Sattentau Q., Thali M., Wu D., Denisova G., Gershoni J., Robinson J., Moore J., and Sodroski J. 1998. CD4-Induced conformational changes in the human immunodeficiency virus type 1 gp120 glycoprotein: Consequences for virus entry and neutralization. J. Virol. 72: 4694–4703.
Tavernier J., Tuypens T., Plaetink G., Verhee A., Fiers W., and Devos R. 1992. Molecular basis of the membrane-anchored and two soluble isoforms of the human interleukin 5 receptor α subunit. Proc. Natl. Acad. Sci. 89: 7041–7045.
Tavernier J., Devos R., Cornelis S., Tuypens T., Van der Heyden J., Fiers W., and Plaetinck G. 1991. A human high affinity interleukin-5 receptor (IL5R) is composed of an IL5-specific alpha chain and a beta chain shared with the receptor for GM-CSF. Cell 66: 1175–1184.
Tavernier J., Van der Heyden J., Verhee A., Brusselle G., Van Ostade X., Vandekerckhove J., North J., Rankin S.M., Kay B., and Robinson D.S. 2000. Interleukin 5 regulates the isoform expression of its own receptor a-subunit. Blood 95: 1600–1607.
Thali M., Moore J.P., Furman C., Charles M., Ho D.D., Robinson J., and Sodroski J. 1993. Characterization of conserved human immunodeficiency virus type 1 gp120 neutralization epitopes exposed upon gp120-CD4 binding. J. Virol. 67: 3978–3988.
Trkola A., Dragic T., Arthos J., Binley J.M., Olson W.C., Allaway G.P., Cheng-Mayer C., Robinson J., Maddon P.J., and Moore J.P. 1996. CD4-dependent, antibody-sensitive interactions between HIV-1 and its coreceptor CCR5. Nature 384: 184–187.
Wilchek M. and Miron T. 2003. Oriented versus random protein immobilization. J. Biochem. Biophys. Methods 55: 67–70.
Wu L., Gerard N.P., Wyatt R., Choe H., Parolin C., Ruffing N., Borsetti A., Cardoso A.A., Desjardin E., Newman W., Gerard C., and Sodroski J. 1996. CD4-induced interaction of primary HIV-1 gp120 glycoproteins with the chemokine receptor CCR-5. Nature 384: 179–183.
Xiang S.H., Kwong P.D., Gupta R., Rizzuto C.D., Casper D.J., Wyatt R., Wang L., Hendrickson W.A., Doyle M.L., and Sodroski J. 2002. Mutagenic stabilization and/or disruption of a CD4-bound state reveals distinct conformations of the human immunodeficiency virus type 1 gp120 envelope glycoprotein. J. Virol. 76: 9888–9899.
Zhang W., Canziani G., Plugariu C., Wyatt R., Sodroski J., Sweet R., Kwong P., Hendrickson W., and Chaiken I. 1999. Conformational changes of gp120 in epitopes near the CCR5 binding site are induced by CD4 and a CD4 miniprotein mimetic. Biochemistry 38: 9405–9416.
Zhukov A., Suckau D., and Buijs J. 2002. From isolation to identification: Using surface plasmon resonance–mass spectrometry in proteomics. PharmaGenomics March/April, 18–28.
Zimmerman M.A., Selzman C.H., Cothren C., Sorensen A.C., Raeburn C.D., and Harken A.H. 2003. Diagnostic implications of C-reactive protein. Arch. Surg. 138: 220–224.
|